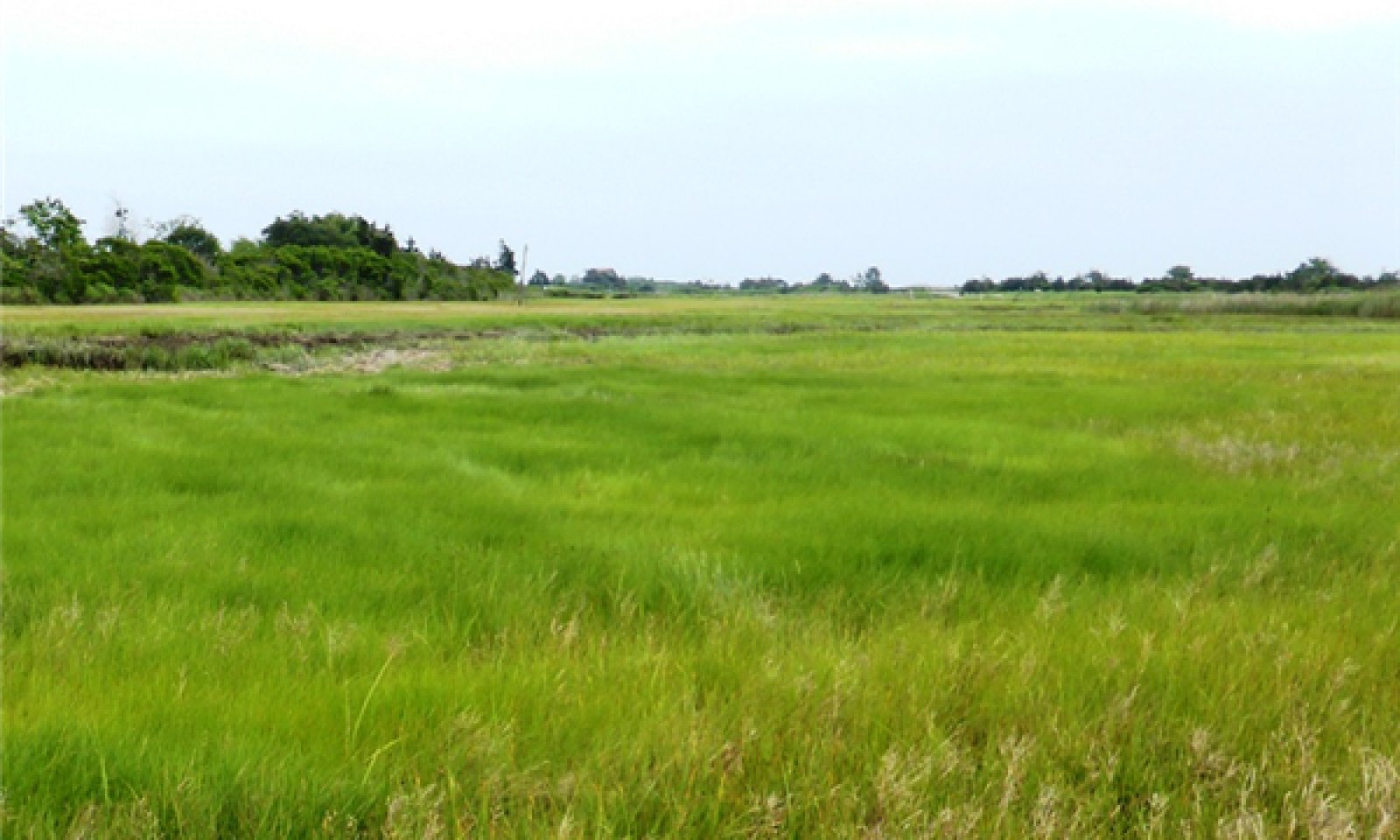

Natural Resources
Conservation Service
Ecological site R144AY002CT
Tidal Salt High Marsh mesic very frequently flooded
Last updated: 10/04/2024
Accessed: 04/12/2025
General information
Approved. An approved ecological site description has undergone quality control and quality assurance review. It contains a working state and transition model, enough information to identify the ecological site, and full documentation for all ecosystem states contained in the state and transition model.
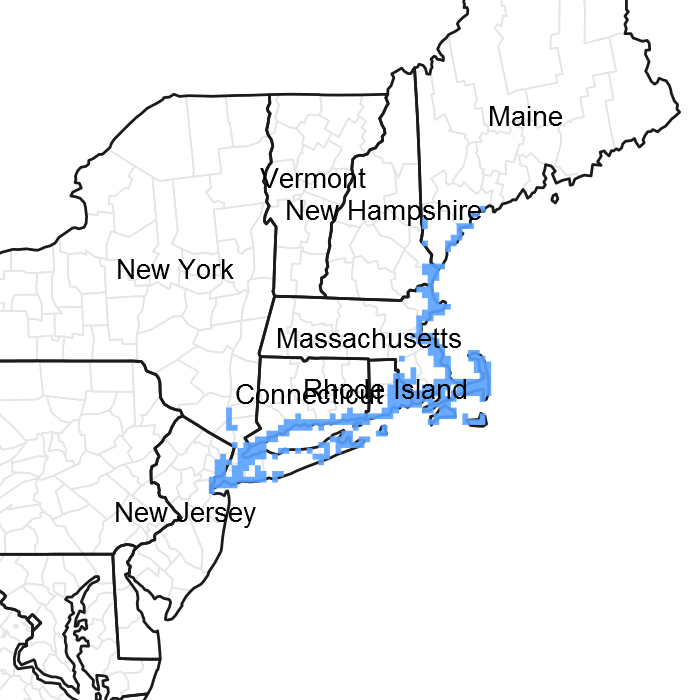
Figure 1. Mapped extent
Areas shown in blue indicate the maximum mapped extent of this ecological site. Other ecological sites likely occur within the highlighted areas. It is also possible for this ecological site to occur outside of highlighted areas if detailed soil survey has not been completed or recently updated.
MLRA notes
Major Land Resource Area (MLRA): 144A–New England and Eastern New York Upland, Southern Part
MLRA 145, Connecticut Valley is based on the concept that glacial Lake Hitchock left finer sediments in the form of varved clays, silts, and fine sands in the middle of a larger and coarser-textured post-glacial environment. The upland areas are mainly comprised of glacial deposits (till and outwash) and/or loess overlying glacial lake sediments, with a mix of recent alluvium and organic residuum in the lower-lying areas directly surrounding the Connecticut River.
MLRA 144A, the New England and Eastern New York Upland, Southern Part is in the New England Upland section of the New England Province of the Appalachian Highlands Division. The area is nearly level to sloping lowlands on the edges of the valley. North to south running trap rock ridges break up the lowlands with hilly, steep areas. Elevation ranges from sea level to 330 feet (100 meters) in the lowlands and from 650 feet to 1,000 feet (200- 305 meters) on ridges.
The tidal marsh ecological sites are located in the south and eastern sections of the MLRA, near the Atlantic Ocean and Long Island Sound coasts and also near the mouths of the major rivers along the coast.
MLRA 149B, Long Island-Cape Cod Coastal Lowland is in the Embayed section of the Coastal Plain Province of the Atlantic Plain Division (Fenneman & Johnson, 1946). It is part of the partially submerged coastal plain of New England. It is mostly an area of nearly level to rolling plains, but it has some steeper hills (glacial moraines). Ridges border the lower plains. Elevation generally ranges from sea level to 80 feet (0 to 25 meters), but it is as much as 410 feet (125 meters) in a few areas.
The tidal marsh ecological sites are located throughout the MLRA, near the Atlantic Ocean and Long Island Sound coasts.
Classification relationships
1. Phytosociologically:
CT: Salt Marshes - Northern marshelder/Switchgrass (Iva frutescens / Panicum virgatum) community; Switchgrass (Panicum virgatum) medium - tall grasslands; Saltmeadow cordgrass – Inland saltgrass (Spartina patens - Distichlis spicata) community (Metzler, and Barrett 2006).
MA: Estuarine Intertidal: Salt Marsh (Swain and Kearsley 2012)
ME: Tidal Marsh Estuary Ecosystem includes: Salt-hay Saltmarsh and Mixed Saltmarsh (Gawler and Cutko 2010).
NJ: Tdal Marsh Complex (Breden 1989)
NH: High Salt Marsh, Marsh elder shrubland, Salt pannes and pools (Sperduto and Nichols 2012)
NY: Salt shrub, High salt marsh, Salt panne (Edinger et al. 2002)
RI: Salt Marsh System, includes High salt marsh; Salt scrub; and Salt panne (Enser et al. 2011).
NatureServe:
CEGL006848 Iva frutescens / Spartina patens Shrubland,
CEGL006006 Spartina patens - Distichlis spicata - (Juncus gerardii) Herbaceous Vegetation
2. Cartographically:
US Forest Service ECOMAP in Keys, J. A. et al. 1995. Ecological Units of
the Eastern United States - First Approximation (Map 1:3,500,00 + Booklet) US Department of Agriculture Forest Service, Atlanta, GA
221A-Lower New England Section
221Aa Boston Basin Subsection
221Ab Cape Cod Coastal Lowland and Islands Subsection
221Ac Narragansett-Bristol Lowland and Islands Subsection
221Ad Southern New England Coastal Lowland Subsection
221Ai Gulf of Maine Coastal Plain Subsection
221An Long Island Coastal Lowland and Moraine Subsection
Ecological site concept
Salt marshes are coastal wetlands that are alternately flooded and drained by salt water borne by oceanic tides. Salt marshes typically develop in sheltered environments but different geomorphic settings where marshy peat and sediment can accumulate, e.g., estuarine coves, bays, behind barrier beaches, drowned valleys, and at the confluence of major rivers,. As coastal landforms, tidal marshes are low in elevation and generally flat, although they can exhibit changes in microrelief which can affect the type of vegetation. The climate depends upon latitude, but evidently it features a strong maritime influence, producing cooler summers and warmer winters. Tidal marsh vegetation is poorly developed but highly productive. Few plant species can physiologically tolerate the high salinities, but those that do can flourish. The predominant vegetation form in the irregularly flooded zone is the tidal salt “high marsh” appearing in its various vegetation-forms as a meadow, upper border, imperfectly drained panne, and quasi permanent pool. Two additional states occur due to habitat manipulations: a Hydrologically-altered state, and a Tidally restricted state. In addition, there are four crossovers to entirely different ecological sites: Tidal Brackish Wetland ecological site, Coastal Dunelands ecological site, Tidal Salt Low Marsh ecological site, and Filled Non-tidal ecological sites.
Similar sites
R144AY001CT |
Tidal Salt Low Marsh mesic very frequently flooded |
---|
Table 1. Dominant plant species
Tree |
Not specified |
---|---|
Shrub |
Not specified |
Herbaceous |
(1) Spartina patens |
Physiographic features
This ecological site occupies the lowest portions of the terrestrial landscape bordering the coast as a narrow intertidal zone primarily composed of organic matter. A water table is found within a few centimeters of the soil surface due to tidal flooding which occurs about twice daily, during the high tides. Runoff or groundwater discharge may be recieved at this physiographic site from surrounding uplands.
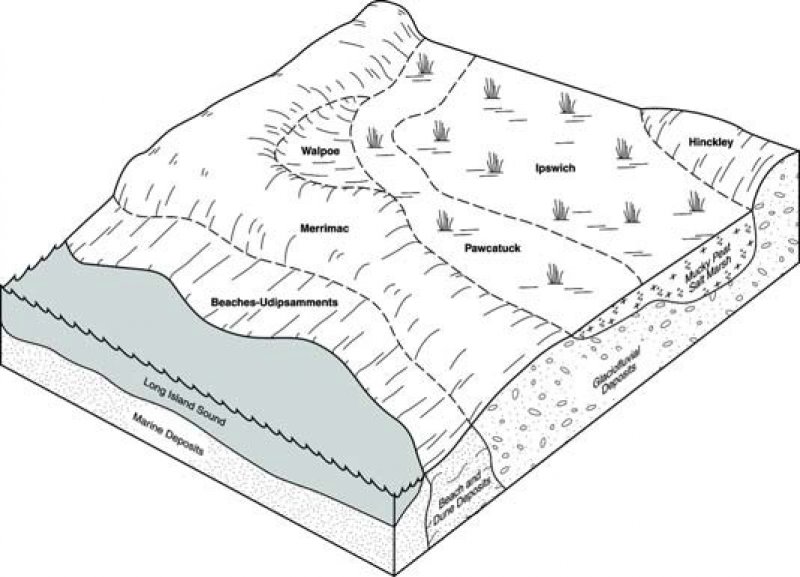
Figure 2. Salt Marsh Block Diagram
Table 2. Representative physiographic features
Landforms |
(1)
Coastal plain
> Salt marsh
(2) Lowland > Tidal marsh |
---|---|
Runoff class | Negligible |
Flooding duration | Extremely brief (0.1 to 4 hours) to very brief (4 to 48 hours) |
Flooding frequency | Frequent to very frequent |
Ponding duration | Very brief (4 to 48 hours) to very long (more than 30 days) |
Ponding frequency | Frequent |
Elevation | 26 ft |
Slope |
Not specified |
Ponding depth | 10 in |
Water table depth | 6 in |
Aspect | Aspect is not a significant factor |
Climatic features
The Koppen-Geiger climate classification of the area in which this MLRA occurs varies between Dfb (Warm-summer humid continental) in the North, and Dfa (Hot-summer humid continental) in the southern portion of the MLRA. Precipitation is usually uniformly distributed throughout the year. Near the coast, however, it is slightly lower in summer. Precipitation is slightly higher in spring and fall in inland areas. Rainfall occurs as high-intensity, convective thunderstorms during the summer. Thunderstorms occur on about 20 days each year, and most occur in July. However, even with a temperate moist climate, normal fluctuations in regional weather patterns can lead to periods of dry weather. The last severe droughts in the Northeast occurred 2000, 2001-2002, 2005, 2007-2008, 2010 and again in 2012, while extreme droughts have not occurred since 2002 (NDMC 2014). During the winter, most of the precipitation occurs as moderate-intensity storms (northeasters) that produce large amounts of rain or snow. The freeze-free period increases in length to the south.
The Atlantic hurricane season runs from June 1st to November 30th. The estimated return period for hurricanes passing within 50 nautical miles of this area of coastal New York and New England ranges 13-43 years with an average of 22.3 years. The return period for major (i.e. Category 3 or greater) hurricanes ranges 52-180 years with an average of 88.0 years. From 1950-2011, these coastal counties have had four Category III hurricane strikes recorded in 1954 (2), 1960 and 1985. They have had one Category II hurricane strike in 1991 and one Category I hurricane strike in 1976 (NOAA NWS 2013).
Future projections indicate that greenhouse warming will cause the globally averaged intensity of tropical cyclones to shift towards stronger storms, with intensity increases of 2–11% by 2100 (Knutson et al 2010).
Table 3. Representative climatic features
Frost-free period (characteristic range) | 131-165 days |
---|---|
Freeze-free period (characteristic range) | 154-206 days |
Precipitation total (characteristic range) | 46-48 in |
Frost-free period (actual range) | 119-178 days |
Freeze-free period (actual range) | 133-213 days |
Precipitation total (actual range) | 44-49 in |
Frost-free period (average) | 148 days |
Freeze-free period (average) | 179 days |
Precipitation total (average) | 47 in |
Figure 3. Monthly precipitation range
Figure 4. Monthly minimum temperature range
Figure 5. Monthly maximum temperature range
Figure 6. Monthly average minimum and maximum temperature
Figure 7. Annual precipitation pattern
Figure 8. Annual average temperature pattern
Climate stations used
-
(1) DURHAM 2 SSW [USW00054795], Durham, NH
-
(2) PLYMOUTH MUNI AP [USW00054769], Carver, MA
-
(3) WESTERLY STATE AP [USW00014794], Westerly, RI
-
(4) BRIDGEPORT SIKORSKY MEM AP [USW00094702], Stratford, CT
-
(5) TETERBORO AP [USW00094741], Moonachie, NJ
Influencing water features
This ESD occurs on tidal marshes near open water with saturated soil conditions year round. The floodwaters are tidal estuarine.
Very poorly drained
Water is removed from the soil so slowly that free water remains at or very near the surface during much of the growing season. Internal free water occurrence is very shallow and persistent or permanent. Unless the soil is artificially drained, most mesophytic crops cannot be grown. The soils are commonly level or depressed and frequently ponded. In areas where rainfall is high or nearly continuous, slope gradients may be greater.
Wetland description
National Wetland Classification (Cowardin et al., 1979):
System: Estuarine
Subsystem: Intertidal
Class: Unconsolidated Bottom, Aquatic Bed, Emergent
Subclass: Cobble-Gravel, Sand, Mud, Algal, Rooted/Floating Vascular, Organic, Persistent/Non-persistent, Phragmites australis
Water Regime: Regularly Flooded
Soil features
This ecological site is represented by soils in the Histosols and Entisols soil orders. Major soil series for this ecological site are Ipswich, Westbrook, and Transquaking. Depth of organic material is variable.
These soils have a mesic soil temperature regime, an aquic soil moisture regime, and mixed mineralogy (Soil Survey Staff, Official Series Descriptions, available online). They have histic epipedons that are shallow to deep to a mineral material (alluvium, glacial till, outwash, or marine deposits) and generally have a sandy or sandy skeletal or a coarse-loamy particle size class when applicable. There does not seem to be a correlation between depth to mineral material and vegetative community type.
A seasonal high water table (SHWT) is at the surface due to tidal flooding. The soil series associated with this ecological site are interpreted to be poorly drained according to Connecticut drainage class standards. Saturated hydraulic conductivity in the soil material is moderately high to very high (Soil Survey Staff, 2013).
Soils associated with this ecological site are formed in organic material deposited over mineral till, outwash, alluvium, or marine deposits. The organic material is derived mainly from native herbacious tidal marsh species, but has been found to be woody with depth as a relict of post-glacial sea level rise. Though most of the material is composed of organic material which generates acids as it breaks down, the salts and minerals deposited by the ocean keep the pH and EC values values high. These unique chemical properties along with sustained periods of saturation are what allow the Spartina species and other salt marsh vegetation to out-compete other species and give the marshes their distinct community banding.
Table 4. Representative soil features
Parent material |
(1)
Herbaceous organic material
(2) Fluviomarine deposits |
---|---|
Surface texture |
(1) Mucky |
Family particle size |
(1) Loamy |
Drainage class | Very poorly drained |
Permeability class | Slow to moderate |
Depth to restrictive layer | 72 in |
Surface fragment cover <=3" | Not specified |
Surface fragment cover >3" | Not specified |
Available water capacity (Depth not specified) |
18 in |
Electrical conductivity (0-40in) |
2 – 60 mmhos/cm |
Soil reaction (1:1 water) (0-40in) |
5.1 – 8 |
Subsurface fragment volume <=3" (Depth not specified) |
5% |
Subsurface fragment volume >3" (Depth not specified) |
Not specified |
Ecological dynamics
Preface: Different classes of tidal wetlands due to changes in salinity and tidal position
Salinity.
Situated between the land and the sea, tidal wetlands are defined in terms of salinity levels and by the vertical extent of the tides. Tidal wetlands include all wetlands subject to the influence of the tides yet vary in salinity, ranging from undiluted seawater to freshwater derived from inland sources such as rivers, runoff, and groundwater seeps. These broad variations in salinity create an intergrading sequence of tidal wetlands ranging from: (1) salt tidal marshes, to (2) brackish tidal wetlands, to (3) freshwater tidal wetlands. The relationship between salinity and wetland class can be approximated by Cowardin et al. (Cowardin et al., 1979; Theve, 2013). (In practice, the term “halinity” is sometimes used in place of salinity to denote ocean salts dominated by halide, NaCl. The units may be expressed in parts-per-thousand [ppt] or virtually equivalent Practical Salinity Units [PSU] (UNESCO, 1981) or commonly expressed as deciSiemans-per-meter [dSm-1]. An approximate conservative conversion is 1 dSm-1 = 0.640 ppt at 25 °C.) Salt marshes typically exhibit salinity levels greater than 18 ppt (28.1 dSm-1), considered polyhaline, 18 - 30 ppt (28.1- 46.9 dSm-1) and levels greater than 30 ppt (46.9 dSm-1), especially in situations where surface evaporation can concentrate salts. The salinity levels of brackish wetlands are more dilute ranging from 0.5 - 18 ppt (0.8 – 28.1 dSm-1), considered oligohaline, 0.5 – 5 ppt (0.8 – 7.8 dSm-1), to mesohaline, 5 – 18 ppt (7.8 – 28.1 dSm-1). Freshwater tidal wetlands exhibit salinity levels of 0.5 ppt (0.8 dSm-1) or less. Salt and brackish tidal marshes are considered estuarine habitats, and are directly influenced by tidal flooding. Salt marshes are found in sheltered, depositional areas located in coves, embayments, and behind barrier beaches. Brackish marshes exist either along landward seeps or drainageways of rivers and streams where fresh and saline waters mix. Freshwater tidal wetlands, while still physically affected by tidal forces, are beyond the reach of the salt front. Lacking salinity, freshwater tidal habitats are sometimes considered riverine habitats (Cowardin et al., 1979), yet other sources consider all tidal wetlands as estuarine (Odum et al., 1984; Odum, 1988). Freshwater tidal and brackish tidal wetlands are optimally developed along the lower reaches of large river systems with low gradients near the confluence with the sea. Furthermore, the floristic dissimilarities between these three classes of tidal wetlands provide a suitable justification for separate Ecological Sites.
Tidal position.
In the simplest terms, each class of tidal wetlands can be separated into two broad zones in reference to the vertical extent of the tides, 1) the “regularly” flooded zone (flooded daily on average); and 2) the “irregularly” flooded zone (flooded less than daily, i.e., only with spring tides). When projected onto the marsh profile these tidally influenced zones are commonly referred to as the “low” marsh (below Mean High Water, MHW) and the “high” marsh (above MHW). However, for the purposes of these ecological site descriptions, only in the case of the salt marsh, are the high marsh and the low marsh treated separately as two different ecological sites. While, brackish and freshwater tidal sites also exhibit similar shifts in plant distribution along the intertidal profile, the geographic extent is very limited, hence the ecological sites of brackish tidal wetlands and freshwater tidal wetlands are considered each in their entirety, aggregating a complex of vegetation-forms over the entire tidal profile.
Tidal salt high marshes of the northeastern United States
The ecological dynamics of the northeastern salt marshes are complex. Most plants have a very limited ability to grow in saline habitats except a small number of specially adapted plants, called “halophytes” (Flowers et al., 1986; Aslam et al., 2011). Furthermore, these same plants are also subjected to increasing physical harshness (decreasing redox potential and increasing salinity) associated with tidal flooding (Nichols, 1920; Miller and Egler, 1950; Niering and Warren, 1980; Bertness and Ellison, 1987). The mosaic pattern of vegetation across salt marshes of the northeast appears roughly in zones due to the limiting influence of physical factors, such as tidal flooding and salinity, and additional effects of both negative and positive biotic interactions among species, like competition and facilitation, respectively (Bertness, 1991a; b; Bertness and Shumway, 1993; Bertness and Hacker, 1994; Bertness and Leonard, 1997; Zhang and Shao, 2013). In addition, superimposed on this complex gradient are several disturbances to the high marsh including: relative sea-level-rise, waterlogging, salt stress, wrack, major storm events, ice-scouring/rafting, and herbivory. Furthermore, additional agents of salt marsh vegetation change are attributed directly or indirectly to human actions, including: accelerated sea-level rise, temperature warming, filling, ditching and draining, open-water-marsh-management (OMWM) and integrated-marsh-management (IMM), alteration of tidal exchange by road crossings, impoundment dikes, culverts, tide gates and other structures, fires, eutrophication, sudden vegetation “dieback”, and consumer control.
Biotic interactions: stress-tolerance / competition trade-off and disturbance-driven facilitation
Besides coping with the changing physical limitations of the intertidal gradient by way of stress and disturbance, plants also experience biotic interactions: competition or facilitation (Bertness and Shumway, 1993; Bertness and Callaway, 1994; Zhang and Shao, 2013).
Competition.
Ecological trade-offs between competition and stress-tolerance are opposing species strategies (Grime, 2006) that explain much of the salt marsh zonation. Since competition is often greatest where stress is minimal, salt marsh species are differentially limited landward by competition and seaward by physical harshness (Bertness, 1991a; b). This accounts for the competitive hierarchy of species observed in the typical northeastern salt marsh (reference state): the woody shrub, Iva frutescens, dominates the terrestrial border and competitively displaces Juncus gerardii to the upper reaches, which displaces Spartina patens to the meadow, which in turn displaces Spartina alterniflora to the low marsh. The same species are ranked in reverse for stress tolerance.
Facilitation.
Furthermore, the vegetation pattern is also the result of secondary succession following disturbance-driven facilitation (Bertness and Shumway, 1993). Facilitation is a positive interaction that occurs when a species ameliorates stress, making the environment more favorable for other, successive species (Connell and Slatyer, 1977; Bruno et al., 2003). Disturbance-generated bare patches and slight depressions known as “pannes” on the high marsh become hypersaline and or waterlogged. The initial colonizers (referred to as foundation species (Angelini et al. 2011)) are stress-tolerant forbs, such as Salicornia spp., or Spartina alterniflora or sometimes Distichlis spicata. The shade cast by these plants that limit salt accumulation, and the accretion of living peat that aerate the rhizosphere, are plant-induced processes that ameliorate the physical harshness of the sites, which can then lead to the succession of the less stress-tolerant competitive dominants, Spartina patens or Juncus gerardii (Bertness and Shumway, 1993; Bertness and Hacker, 1994; Bertness and Leonard, 1997).
In summary, facilitation is common in secondary succession under increased environmental stressors, but competition dominates under more benign physical conditions (Bertness and Shumway, 1993).
Relative Sea-level Rise
Under natural conditions, salt marsh elevations are generally near or approaching a dynamic equilibrium relative to rising sea level (Friedrichs and Perry, 2001; Morris et al., 2002). Historically, marsh-building has occurred both landward and seaward in southern New England during times of slow [historic] rates of sea-level rise at approximately 1.0 mm•yr-1 (Nixon, 1982; Donnelly et al., 2004) and marshes may appear to continue building during moderate rates of sea-level rise at approximately 2.5 mm yr-1 (Bricker-Urso et al., 1989; Donnelly et al., 2004; NOAA/NOS/CO-OPS, 2014). However, there is a concern that the accelerated rates of sea-level rise may eventually impose an upper limit or tipping point beyond the ability of coastal marshes to build and keep pace with sea-level rise (Bricker-Urso et al., 1989; Kirwan et al., 2010). (More details follow in the section on accelerated sea-level rise).
Waterlogging
Waterlogging within the marsh profile results from impediments to soil drainage due to changes in surface micro-relief (Miller and Egler, 1950; Niering and Warren, 1975; Nixon, 1982; Ewanchuk and Bertness, 2004). The surface of the high marsh is periodically interrupted with low depressions consisting of inadequately drained pannes, secondary pannes, permanently flooded pools and excavated pools. Waterlogging on the salt marsh generates a physically stressful habitat with anaerobic or anoxic (oxygen-deficient) conditions that challenge plant establishment, growth, and survival (Ewanchuk and Bertness, 2004; Tiner, 2013). Besides waterlogging, cycles of flooding and evaporative exposure create extremes in salinity, further contributing to the harshness of these habitats. Hence, extremely stressful pannes may be devoid of vegetation or covered with a mat of cyanobacteria or filamentous green alga, Cladophora gracilis. Intolerant to physical stresses, the main turf-forming graminoids (i.e., grass or grass-like plants), Spartina patens and Juncus gerardii are physically excluded. However, stress-tolerant forbs and short-form Spartina alterniflora are able to withstand some waterlogging and act as pioneering plants which persist for a while without competitive displacement (Bertness, 1991a, 1992; Ewanchuk and Bertness, 2004). These “safe-sites” for these species (Harper, 1977) persist as long as cycles of flooding and exposure maintain or renew the waterlogged nature of the site. However, these safe-sites may only serve as a temporary refuge. Often the mere occupation of a site by plants will have an ameliorating effect. Aeration of the rhizosphere and elevational accretion are plant-induced site modifications that can facilitate re-invasion by turf-forming graminoids (Bertness and Shumway, 1993; Bertness and Hacker, 1994; Tiner, 2013; Zhang and Shao, 2013).
Cyclical changes in Metonic high tides (18.6 year cycle) can affect plant life with increased tidal flooding frequency and duration (Tiner, 2013). The resulting prolonged waterlogging may instigate detrimental feedback, where limits to plant growth further limit vertical accretion which exacerbates waterlogging, etc. (Friedrichs and Perry, 2001). Waterlogging can be produced by relative sea-level rise, marsh subsidence, occluded creeks, micro-levees, and or reduced sediment load (Niering and Warren, 1975; Friedrichs and Perry, 2001; Ewanchuk and Bertness, 2004; Adamowicz and Roman, 2005). Waterlogging has also been implicated as a common stress associated with dieback (Smith and Carullo, 2007; Alber et al., 2008). The prevalence of severe waterlogging, i.e., drowning, may be symptomatic of accelerated sea-level rise (Warren and Niering, 1993; Hartig et al., 2002) and crossover to a different ecological site entirely (e.g., the regularly flooded, “tidal salt low marsh”).
Salt stress (salinity extremes)
Salinity in salt marshes can be quite variable (Tiner, 2013). Dilution can occur during freshwater inputs from high precipitation events producing upland runoff and groundwater seepage, and concentration can occur during cycles of tidal flooding and evaporation. In general, salinity gradients produced by these processes contribute to the physical stress gradient intensifying across the marsh from upland to open water. In addition, hyper-saline conditions can occur anywhere in disturbed bare patches or pannes (Nichols, 1920; Miller and Egler, 1950; Niering and Warren, 1980). Most plants have a very limited ability to grow in saline habitats except a small number of specially adapted plants, called “halophytes” (Flowers et al., 1986; Aslam et al., 2011). Accordingly, most salt marsh plants tolerate moderate salinity, but even fewer plants can withstand the elevated salinity of bare patches and pannes ranging from 35 to 50 ppt (54.7 – 78.1 dSm-1) and above (Niering and Warren, 1980). The ability to withstand conditions such as salt stress (and waterlogging), enables stress-tolerant plants such as Salicornia spp., stunted Spartina alterniflora, and other forbs a chance to escape immediate competition from the less stress-tolerant turf-forming graminoids, Spartina patens and Juncus gerardii. However, these refugia are often temporary. As these plants fill the site, their shade will limit evaporation and salt accumulation, thereby reducing physical stress, and ultimately making the site suitable for less stress-tolerant but more competitive plants (Bertness and Shumway, 1993; Zhang and Shao, 2013).
Predicted climate warming (IPCC, 2013b) may create hyper-saline conditions, ranging in effects from salt pannes to greater high marsh facilitation and dominance by turf building graminoids (Gedan and Bertness, 2009).
Wrack
The most common natural disturbance in Northeastern US salt marshes is the stranding of wrack. Deposited by extreme high and storm tides, the smothering action of wrack kills vegetation and renders the patch bare. In Northeastern US tidal marshes, wrack is made up mostly from decaying litter of Spartina alterniflora, and to lesser extent of Zostera marina (eelgrass). Wrack disturbance covers 6 to 15 percent of the high marsh surface annually (Hartman, 1984; Bertness and Ellison, 1987), albeit, the areas actually damaged by wrack are usually less if the wrack is not persistent (Valiela and Rietsma, 1995). The extent and distribution varies considerably over the marsh, but persistent wrack does accumulate more in the upper border (Miller and Egler, 1950; Bertness and Yeh, 1994), providing opportunities for Iva frutescens spp. oraria (maritime marsh elder) establishment. The damage facilitated by the smothering action of wrack is also a mechanism for secondary panne formation, and provides colonization opportunities for Distichlis spicata (saltgrass) and competitively subordinate plants (Brewer et al., 1998). Forbs frequently establish by seeds, whereas Distichlis spicata colonizes vegetatively (Bertness and Ellison, 1987).
Ice scouring and ice rafting
With increasing latitude, more northern salt marshes are progressively exposed to the effects of two different types of winter ice disturbances, ice scouring and ice rafting, which can trigger vegetation change. Winter ice damages low marsh, but ice also can impact the high marsh to some degree. Ice scouring is not as frequent as wrack disturbance, but generally more severe, leaving behind ice scars and hollows that persist for years where the marsh peat was removed or plucked (Ewanchuk and Bertness, 2003). Ice rafted debris was noted as a major source of sediment in Maine tidal marshes (Wood et al., 1989). Localized erosion and deposition from ice disturbances, enhance the surface microtopography and create bare areas that develop secondarily into pannes and pools.
Major storm events
Coastal storms have been associated with both marsh erosion and marsh accretion. In addition, subsurface effects such as subsidence can result (Cahoon, 2006). Changes in marsh topography and position have marked influence on the distribution of marsh plants. Direct wave erosion (scouring) of the marsh surface happens during periods of extreme high tide, but impacts are minimized due to the damping of flow through marsh vegetation. More commonly, direct erosion occurs along the creeks crossing the high marsh where strong tidal currents can undermine banks or along seaward bayfronts where there is heavy wave action (Friedrichs and Perry, 2001). Instead, storm related erosion is most often associated with events of ice-scouring and ice rafting in northern New England (Ewanchuk and Bertness, 2003) and the marsh-smothering aftermath of wrack deposition (Niering and Warren, 1980; Bertness, 1992) that leads to the creation of waterlogged pannes and pools which may degrade the interior of the marsh. Conversely, most sediment deposition in tidal marshes are attributed to coastal storm events (Harrison and Bloom, 1977; Stumpf, 1983; Gedan et al., 2009). During extreme high tides and storm surges that overtop the marsh are called “overwash”, and loads of sediment deposited are called “washovers”. The washovers often form progressive bands parallel to the marsh edge, potentially changing the microtopography of the marsh profile. If severe enough, washovers of sufficient material and magnitude, can convert the tidal marsh to “dunelands”, an entirely different ecological site. Otherwise, if the storm surge is more like a spring tide, deposition are controlled by the creeks, which scour creek and ditch bottoms and build levees (Friedrichs and Perry, 2001). The sedimentary record in a Rhode Island marsh revealed at least 7 hurricane-magnitude storms within the past 700 years (Donnelly et al., 2001). Climate change scenarios predict that the intensity of major storm activity will increase in the North Atlantic (IPCC, 2013a).
Herbivory
Herbivory is not usually considered a significant factor in the structuring of saline high marsh plant communities in the northeast. Although, pronounced insect herbivory has been reported in patches of Salicornia sp. (Ellison, 1987) and seed set reduction of 50 percent upon common marsh perennials (Bertness and Ellison, 1987). Yet, herbivory is more important in the low marsh, dominated by Spartina alterniflora, where triggered by indirect effects of human disturbance, significant grazing impacts have been noted by geese, snails, and crabs and insects (see later section about effects of Consumer Control).
Accelerated Sea Level Rise – vertical accretion and lateral migration
Stratigraphic evidence and tide gage information has indicated that sea-level rise in New England accelerated during the last century from about 1.0 mm yr-1 (Nixon, 1982; Donnelly et al., 2004) to a rate of approximately 2.6 mm yr-1 (1938 – 2006, USGS New London tide gage no. 8461490). The rate may be increased to 4.5 mm yr-1 if adjusted for the latest gage data trend segment from 1987-2013 (Boon, 2012; Sallenger et al., 2012). AR5, the Fifth Assessment Report, (IPCC, 2013b) predicts that the rate of global mean sea level (GMSL) rise may conservatively increase to 4.5 mm yr-1 by mid-century and continue to accelerate to 11 mm yr-1 or even as high as 16 mm yr-1 by the end of the 21st century in response to a decadal warming trend ranging from 0.08 to 0.14 °C. These recent trends and projections in accelerated sea level rise due to climate change (global warming ) (Varekamp and Thomas, 1998; Boon, 2012; IPCC, 2013b) have caused concern about (1) whether vertical salt marsh accretion can keep pace (Reed, 1995; Kirwan et al., 2010), (2) whether the horizontal development of marshes has sufficient neighboring space upon which to laterally migrate. Any significant changes in the vertical or lateral extent of the tidal marsh can lead to a state change in the vegetation.
Vertical accretion.
Vertical stability of the tidal marsh’s surface elevation is achieved when wetland surface elevation gains are approximately the same rate of sea level rise. Elevation gains are driven by the accumulation of organic matter (the persistent buildup of belowground vegetation growth and litter, or soil O- horizons) and the accumulation of inorganic sediment, minus any losses attributed to erosion, subsidence (i.e., decomposition, and compaction) (Morris et al., 2002; Cahoon et al., 2006; Fagherazzi et al., 2012). To precisely document vertical processes, a network of “Surface-Elevation Table and Marker Horizon” (SET-MH) set-ups exist throughout New England and elsewhere (Cahoon et al., 1999, 2006). The SET-MH results are used as a measure of marsh resiliency or vulnerabilityassociated with rising sea level and to document changes to vegetation states and to ecological sites. Depending on the location and geomorphic setting, the contribution of vertical processes of marsh accretion varies. In New England, peaty organic matter accumulation dominates vertical accretion (Bricker-Urso et al., 1989; Turner, 2011). Organic matter contributes more significantly to vertical marsh building on a volume basis than sediment accumulation, building “elevation capital” to buffer increase in sea level (Cahoon and Guntenspergen, 2010). Predictions of warmer temperatures and longer growing season may also enhance plant productivity and decomposition with the net effect of adding gains to offset marsh losses to rising sea levels (Charles and Dukes, 2009; Kirwan and Megonigal, 2013). However, as sea level continues to rise, organic matter accrual is eventually limited by “drowning’ when increasing tidal flood levels no longer allow plant growth (Morris et al., 2002; Kirwan et al., 2010). Yet increasing flood levels relative to lowered marsh elevation favor an increase in the rate of inorganic sediments (Friedrichs and Perry, 2001; Morris et al., 2002) which now become more important than organic matter accrual. (Fagherazzi et al., 2012; Kirwan and Megonigal, 2013). Due to low sediment concentrations, the peaty organic matter-based marshes of the northeastern United States may be less resilient to sea level increases than more sediment laden salt marshes in the southeastern and gulf regions of the United States (Gedan and Bertness, 2010; Weston, 2014). For example, models predict a threshold sea level rise rate of 5 mm yr-1 for the peat marshes in the Plum Island Estuary, MA assuming suspended sediment concentrations remain low (Kirwan et al., 2010). In New England, evidence of submerging marshes is revealed by shifts and disappearance of vegetation currently observed in southweatern Long Island Sound (Tiner et al., 2006), Jamaica Bay (Hartig et al., 2002), and Cape Cod (Smith, 2009). These predictions suggest that once the elevation capital is spent, these high marshes will convert to the “tidal salt low marsh ecological site”.
Lateral migration.
Another aspect of sea level rise is lateral migration, where salt marshes expand into adjacent low lying wetlands, up rivers, and onto uplands. This process, known as “marine transgression”, has continued at varying rates since the last glaciation (Redfield, 1972; Niering and Warren, 1980; van de Plassche, 1991). With accelerated sea level rise, the tidal marshes may appear to be retreating in areas where the low marsh boundaries and vegetation zones are shifting landward (Niering and Warren, 1980; Donnelly and Bertness, 2001) along with the encroaching-landward transgressive edge of the tidal marsh. However, any sea level induced losses can only be offset by transgression if suitable lowlands exist and human barriers for marsh transgression are minimal (Kirwan and Megonigal, 2013). Spatially explicit models showing future marsh migration locales are being used for insight into the into coastal planning and resource management (Fagherazzi et al., 2012). Sea Level Affecting Marshes Model (SLAMM) is a spatial GIS model that simulates the dominant processes involved in wetland conversions and shoreline modifications during long-term sea level rise (Mcleod et al., 2010). Distributions of wetlands are predicted under conditions of accelerated sea level rise, and results are summarized in tabular and graphical form; http://warrenpinnacle.com/prof/SLAMM/index.html. The Nature Conservancy’s Coastal Resilience Project includes a GIS tool that identifies coastal areas suitable for migration, as well as potential barriers to migration, and the relative importance of different marshes based on their potential to provide ecosystem services. In New England, the migration potential has been mapped for marshes in Long Island and Connecticut http://coastalresilience.org/geographies/new-york-and-connecticut ; http://maps.coastalresilience.org/nyct/#, (Hoover et al., 2010).
Temperature warming
In addition to driving accelerated sea level rise (IPCC, 2013b), temperature warming more subtly affects the composition and distribution of salt marsh vegetation directly and by extending growing season. In spite of waterlogging due to rising sea levels (Warren and Niering, 1993), higher temperatures, can affect greater evaporation, cause hypersalinity, and create drier conditions, as demonstrated by latitudinal and experimental studies (Pennings and Bertness, 1999; Bertness and Ewanchuk, 2002; Charles and Dukes, 2009; Gedan and Bertness, 2009). Depending on the rate of sea level rise and the marsh elevation, two scenarios are likely: 1) greater cycles of flooding and evaporation generating more forb or salt pannes at the expense of high marsh meadow (Warren and Niering, 1993; Pennings and Bertness, 1999; Kirwan and Megonigal, 2013), or 2) generating marsh meadow , at least temporarily, as the evapotranspiraton and productivity of forbs, lessen the stress of waterlogging and salinity, only to be replaced by facilitating turf-building graminoids such as Spartina patens (Gedan and Bertness, 2009, 2010).
Filling
Filling coastal marshes for land reclamation projects ranks among the most radical and greatest impact because the process almost always results in permanent habitat loss and conversion to a variety of “non-tidal and upland ecological sites”. Losses due to dredge and fill operations were common to improve navigation and expand seaports and marinas (Rozsa, 1995; Tiner, 2013). Other more subtle impacts include incidental fill and indirect hydrologic impacts resulting from road crossings and impoundment dikes that interfere with normal tidal flow causing a decrease in sediment supply to the marsh surface and a decrease in vertical accretion (Kennish, 2001). Anecdotal accounts often state coastal wetland losses at 50 percent (Gosselink and Baumann, 1980). Some tidal marsh assessments have estimated 30 percent loss for the Long Island Sound from 1880 to 1970 (Dreyer et al., 1995) and 37 percent for parts of Maine, New Hampshire, Massachusetts, and Rhode Island (from roughly 150 to 200 years ago until present) (Bromberg and Bertness, 2005). Coastal marsh losses in the greater Boston region is estimated at 81 percent (Bromberg and Bertness, 2005). It may be questionable whether those estimates for coastal salt marsh losses fully account the widespread filling that resulted from expansion of the maritime economy of New England in the mid 1700’s, the industrial revolution in the early 1800’s, and the full extent of coastal development over the last 250 years (Nixon, 1982).
Ditching
Historically, New England salt marshes have been extensively ditched and drained for salt hay farming and mosquito control (Miller and Egler, 1950; Redfield, 1972; Niering and Warren, 1980; Nixon, 1982). Conventional salt marsh haying appeared to have had little effect upon the ecology except promoting Spartina patens over Spartina alterniflora. However, many hayers also “worked” the marshes, meaning ditching and draining the marsh surface to further favor Spartina patens, (Buchsbaum et al., 2009). The most intensive ditching occurred in the 1930s, by the Civil Works Administration and Works Progress Administration, for the purposes of mosquito control and employment (Rozsa, 1995; Gedan et al., 2009). The type of ditch network most commonly excavated in New England was a pattern of successive straight parallel ditches running from upland to open water (sometimes called grid ditches even if cross-ditching was not evident). The intent of ditching was to decrease mosquito populations at the source by removing standing water where mosquito larvae develop; a practice that has since been judged of questionable or minimal benefit (Nixon, 1982; Tonjes, 2013). Currently, 94 percent of New England marshes have been ditched (Crain et al., 2009). Historically, pannes and pools were more common features on the high marsh and thus the scarcity of salt marsh pools today is a legacy of intensive ditching for mosquito control and salt hay operations (Ewanchuk and Bertness, 2004; Adamowicz and Roman, 2005).
Ditching is a major hydrological alteration to the salt high marsh, but less so to the low marsh. Ditches circumvent the natural drainage system with a network of shortcuts that increase the marsh surface area enhanced by tidal flooding and drainage that would not ordinarily be affected by tidal flooding except for extreme highs (Tonjes, 2013). The effects of disrupting the normal tidal fluctuations are dependent on the site tide range and density of the ditch network (Friedrichs and Perry, 2001). Yet there are some generalities, for example, newly cut ditches lessen waterlogging with peat drainage up to five meters away from ditches (Crain et al., 2009). By altering hydrologic conditions, ditching has pronounced effect on the vegetation pattern. Ditching impacts the surface drainage and microtopography plus drains the marsh pools, eliminating Ruppia maritima (Miller and Egler, 1950; Adamowicz and Roman, 2005). New ditches will ameliorate waterlogging favoring more competitive turf species such as Spartina patens and Juncus gerardii over forbs and Spartina alterniflora (Bertness, 1991a, 1992; Ewanchuk and Bertness, 2004). Just as creeks do, ditches also direct sedimentation to form levees that impede and convey surface drainage (Miller and Egler, 1950; Warren and Niering, 1993). Once large expanses of high marsh dominated by Spartina patens now appear as fragmented wetlands growing Iva frutescens and potentially invasive Phragmites australis on the levees that have built naturally by deposition or on levees artificially constructed of persistent spoils deposited ditchside or creekside. Ironically, the legacy of parallel ditching has often created large very pannes of stunted Spartina alterniflora and Salicornia spp. sometimes filling the inter-ditch area (Miller and Egler, 1950; Niering and Warren, 1980; Barrett and Niering, 1993). Ditches also create low marsh habitats supporting Spartina alterniflora along ditch banks and in aggraded ditches (Miller and Egler, 1950). Hence, ditches have expanded and accelerated sudden vegetation die-backs by increasing the area of susceptible low marsh habitat (Alber, Swenson, Adamowicz, & Mendelssohn, 2008; Coverdale, Bertness, & Alteiri, 2013). Enhanced ditch circulation translates to potential nutrient enrichment and eutrophication (Koch and Gobler, 2009; Tonjes, 2013) favoring either Spartina alterniflora or the invasive Phragmites australis in less waterlogged, less saline situations (Amsberry et al., 2000; Tiner, 2013).
Fire
Fire may play an important role in some coastal marsh ecosystems (Nyman and Chabreck, 1995), but the significance of fire is uncertain in high marshes of the northeastern US. Given the importance of early native American fires, speculation suggests that high marshes may have been burned (Miller and Egler, 1950). Today, accidental fires are more common to Phragmites australis brackish tidal wetlands (Roman et al., 1984).
Eutrophication (nutrient loading)
Eutrophication, particularly nitrogen inputs originating from shoreline development and removal of adjacent vegetation buffers is contributing to shifting changes in the salt marsh vegetation (Silliman et al., 2009; Gedan et al., 2011). Since most salt marsh plants are nitrogen limited, eutrophication disrupts the competitive hierarchy/stress and disturbance gradients associated with the typical zonation of the Northeastern US salt marsh (Emery et al., 2001). This process affects the expansion of Spartina alterniflora into the high marsh displacing Spartina patens and the proliferation of Phragmites australis at the upper boarder displacing Juncus gerardii (Levine et al., 1998; Bertness et al., 2002). Eutrophication is also implicated in reduced marsh accretion and subsidence leading to greater panne formation. Although nitrogen additions can increase aboveground biomass, eutrophication reduces the nutrient seeking belowground root and rhizomatous biomass volume and strength, ultimately leading to plant collapse, and potentially drowning (Valiela et al., 1976; Turner et al., 2009; Gedan et al., 2011). However some nitrogen enriched salt marshes already effected may not exhibit reduced biomass and elevation loss (Anisfeld and Hill, 2012). Eutrophication can trigger consumer control by herbivorous insects which can suppress primary production by as much as 50 percent (Bertness and Silliman, 2008).
Open Marsh Water Management and Integrated Marsh Management
Open water marsh management (OMWM) was a response of managers to address the long-term failures of ditching to more effectively control mosquitoes. The concept of OMWM is to re-create irregularly flooded saltmarsh pools that were eliminated by ditching to provide habitat for larvivorous mosquito-eating fish, notably the mummichog, Fundulus heteroclitus (Rochlin, James-Pirri, Adamowicz, Dempsey, et al., 2012). OMWM semi-tidal runnels (shallow ditches), pools, and larger ponds are created using rotary excavators and the selective plugging of existing ditches, an altogether uncertain methodology (Vincent et al., 2013). OMWM may have less impact on the physical, hydrological, and biological character of the tidal marsh than grid ditching. However, pools created from plugged ditches are not the same as natural pools. OMWM produces greater surface water, lower redox potential, low soil bulk density, and greater subsidence. Species associated with waterlogging are Spartina alterniflora and forb panne species, Triglochin maritimum, Plantago maritima, Atriplex patula, Gerardia maritima, Limonium carolinianum, and Sueda spp. (Vincent et al., 2013).
Integrated marsh management (IMM) is a multi-purposeful approach to salt marsh ecosystem management that uses best management practices of salt marsh restoration while also incorporating various mosquito control methods (Rochlin, James-Pirri, Adamowicz, Wolfe, et al., 2012). The main goal of IMM is to restore or emulate native salt marsh conditions using mostly geomorphic alterations to achieve tidal flow restoration, vegetation management, and aspects of OMWM. However, since IMM an OMWM projects tend to be highly physically disruptive, a systematic program is strongly recommended to first evaluate and always monitor the impacts and benefits to the vegetation (Potente, 2007).
Tidal restriction and exclusion due to causeways, impoundment dikes, culverts, and tide gates
Tidal restriction limits coastal marshes from essential marine tidal influences, which alters the physical and biotic conditions on the marsh leading to change in the vegetation. For example, tidal restrictions like dikes and impoundments are constructed across coastal wetlands and tidal waterways to manipulate coastal marshes for many reasons, e.g., salt haying, tide mill ponds, wildlife management, flood control, or to build transportation causeways, like road crossings (Kennish, 2001). Tidal restrictions may involve adjustable water control structures such as weirs, flap gates, and self-regulating gates. Additionally, tidal restrictions may simply be the result of undersized or inappropriately positioned road culverts (Roman et al., 1984). Berms and dikes that prohibit sheet flow of flood tides across the marsh surface coupled with one-way “flapper” gates are some of the most restrictive structures. Marsh habitats behind constructed dikes are effectively isolated from marine influence and degrade due to reduced accretion, lowered water tables, and reduced salinity. Reduced salinity results in in the invasion of brackish marsh species (Roman et al., 1984) such as cattails, (Typha latifolia and Typha x glauca) and bulrushes (Schoenoplectus spp.) as well as the common reed (Phragmites australis or possibly the native genotype, Phragmites australis ssp. americanus). In extreme situations, if drained, the aerated organic matter will decompose, oxidize pyrite (iron sulfides), and acidify the soil (Portnoy and Giblin, 1997). These acid sulfate soils, also called “cat-clays”, are severely acid enough to prohibit plants and may appear barren. Furthermore, interrupting tidal flow and increasing surface water impoundment not only collapses the marsh but reduces the “elevation capital” which diminishes the ability of the marsh to keep pace with sea level rise and contributes to salt marsh habitat loss over time (Gedan et al., 2009). Tidal restriction is an acute problem in the industrialized northeastern United States and a primary focus of restoration (Burdick and Roman, 2012).
Marsh sudden vegetation “dieback”
Sudden vegetation dieback, SVD, also known as simply “dieback”, is a condition that has been described as the loss or death of emergent vegetation in salt marsh systems. It was originally characterized by standing dead vegetation only, rather than sites that were over-grazed, wracked, ice-sheared, etc. (Alber et al., 2008). While the nature of marsh dieback is clear, the causes of dieback is poorly understood and controversial (Linthurst, 1979; Alber et al., 2008; Holdredge et al., 2009; Smith, 2009; Elmer and Marra, 2011; Anisfeld and Hill, 2012). Marsh dieback is not usually associated with the salt high marsh although exceptions exist on the tidal wetlands of Cape Cod, MA (Smith, 2009). Marsh dieback is a significant issue in the salt low marsh sites impacting Spartina alterniflora.
Consumer Control (subsidized or unchecked herbivory)
Among the impacts to salt marshes attributed to human activity, vegetation losses due to indirect and subtle factors, such as consumer control are less understood. Triggered by human disturbance, herbivory by plant consumers either goes unchecked or gets subsidized, resulting in significant vegetation loss in areas where plant consumers were not historically important (Power, 1992; Pace et al., 1999; Bertness and Silliman, 2008). Examples of consumer control triggered by human disturbance, include significant grazing impacts by geese, snails, and crabs and insects, mostly upon Spartina alterniflora, especially in the low marsh and only occasionally in the high marsh.
Salt marsh eutrophication from enriched discharges and runoff, enhance insect herbivory by grasshoppers, Conocephalus spartinae, and leafhoppers, Prokelisia marginata, reducing the vegetation by nearly 60 percent (Bertness et al., 2008; Sala et al., 2008).
Fueled by fertilizer subsidies to agricultural fields and golf courses, populations of gregarious and migratory geese inflated to nuisance levels. In such situations, snow geese, Chen (Anser) caerulescens, have denuded expansive marshes in the Subarctic (Jefferies et al., 2006) and to a lesser extent southeastern US (Smith and Odum, 1981), and Canada geese, Branta Canadensis, overgraze saltmarshes throughout New England (Buchsbaum et al., 1981; Teal, 1986).
On Cape Cod and perhaps elsewhere, recreational fishing has depleted predatory fish, allowing increases in the populations of the native, herbivorous purple marsh crab, Sesarma reticulatum, that completely denude the high marsh ditches and low marsh of Spartina alterniflora (Holdredge et al., 2009; Altieri et al., 2012; Coverdale, Herrmann, et al., 2013).
The waterlogged condition anticipated with accelerated sea-level rise is expected to heighten the grazing pressure of the long-introduced common periwinkle, Littorina littorea, (Bequaert, 1943) on Spartina alternifora along high marsh creekbanks and the low marsh (Bertness, 1984; Tyrrell et al., 2008).
State Transitions and Ecological Site Transitions
Several transitions occur that transform the typical salt high marsh reference condition. Two are state transitions that result directly from hydrological alteration and tidal restriction. Both states are subject to restoration actions. These state transitions are featured by appropriate numbers at the core of the state-and-transition model and within appropriate sections of the corresponding narrative. Four additional transitions are warranted that result in crossovers to entirely different ecological sites. These are external transitions that lead to, or, lead from, other ecological sites. (Unfortunately, current database limitations do not easily account for levels beyond a state transition. Therefore, an alternative solution is to simply include external transitions in the diagram without numbers or boxes and add a short description in the narrative.) External transitions to other ecological sites are nonetheless important to the ecological dynamics of the salt marsh ecosystem. These external ecological site transitions include highly significant yet commonplace processes or disturbances, and are listed as follows:
Transition to irreversibly Filled ecological sits of various types
The deposition of fill, human-transported material, to the extent of excluding the tides, creates non-tidal and upland ecological sites of various types.
Transition to Coastal Dunelands ecological site
Infrequent storms of severe magnitude create huge surges that deposit large volumes of sand in overwash. The resulting coastal dunelands ecological sites may be un-vegetated or sparsely vegetated with Ammophila breviligulata (American beachgrass), or only occasionally vegetated at lower elevations with Spartina patens (saltmeadow hay).
Transition from Tidal Brackish Wetland ecological site to Tidal Salt High Marsh ecological site.
Increasing flood stress associated with accelerated sea level rise results in the displacement of the brackish tidal wetland, dominated by Panicum virgatum (switchgrass), Typha spp. (cattails), and or Phragmites australis (common reed), by the tidal salt high marsh ecological site. Iva frutenscens ssp. oraria (maritime marsh elder) may also pioneer here.
Transition from Tidal Salt High Marsh ecological site to Tidal Salt Low Marsh ecological site
A shift up and landward in the regularly flooded (flooded daily) intertidal zone associated with accelerated sea level rise results in greater flood stress leading to the displacement of the Tidal Salt High Marsh ecological site to the Tidal Salt Low Marsh ecological site, dominated by Spartina alterniflora (smooth cordgrass).
The ecology of North Eastern tidal wetlands is well studied. Accounts of the vegetation and dynamics reported are derived largely from the literature and augmented by field sampling.
The information contained in this Ecological Site Description (ESD) and State and Transition Model (STM) were developed using historical data, professional experience, and scientific studies. The information presented is representative of a very complex set of plant communities. Not all scenarios or plants may be included. Key indicator plants, animals and ecological processes are described to inform land management decisions.
Additional information for Tidal Salt High Marsh R144AR002CT is based on original field observations and relevé plots sampled. The field work was conducted by the staff of State Soil Office 12-TOL, and NRCS Ecologist. Additional relevé plots were provided courtesy of VegBank, the CT Natural Diversity Database (Natural Heritage Program), and CT Department of Energy and Environmental Protection, Wildlife Division.
State and transition model
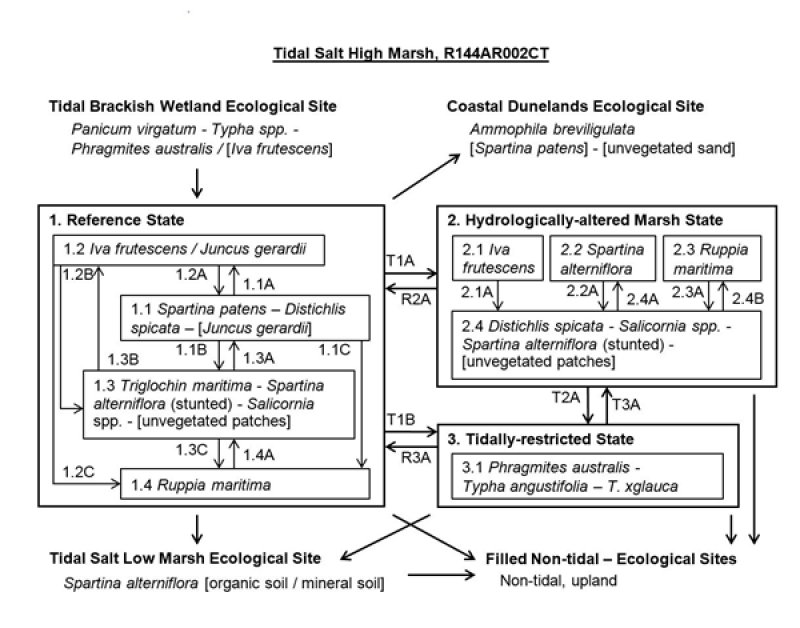
Figure 9. Tidal Salt High Marsh R144AR002CT
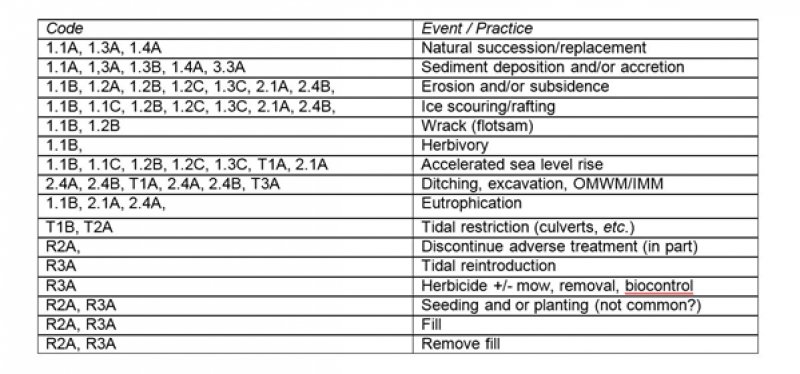
Figure 10. Tidal Salt High Marsh R144AR002CT
More interactive model formats are also available.
View Interactive Models
More interactive model formats are also available.
View Interactive Models
Click on state and transition labels to scroll to the respective text
State 1 submodel, plant communities
State 2 submodel, plant communities
State 3 submodel, plant communities
State 1
Reference
The reference state is a typical tidal salt high marsh of the northeastern United States, characterized as a complex mosaic dominated by (1.1) “salt meadows” of pure and or mixed graminoids: Spartina patens (saltmeadow cordgrass) and Distichlis spicata (saltgrass) and Juncus gerardii (saltmeadow rush). Areas of high marsh with less frequent flooding, at higher elevations and landward reaches, support (1.2) “upper salt meadows and/or shrublands” composed predominantly of Juncus gerardii and Iva frutescens ssp. oraria. (Jesuit’s bark or maritime marsh-elder). The high marsh is further interrupted by (1.3) patches of floristically distinct “pannes”: typified by a sequence of three types, listed in order of increased, but temporary, ponding: (i) shallow forb panne variant typically dominated by Triglochin maritima (seaside arrow-grass), (ii) stunted Spartina alterniflora (smooth cordgrass) – Salicornia depressa (common glasswort or Virginia glasswort) panne variant, and (iii) an unvegetated salt panne variant. Another occasional high marsh feature is (1.4) permanently flooded pools of Ruppia maritima (widgeongrass or beaked ditchgrass). Note that some transitions away from the reference site lead to different states and still other more severe transitions occur that transform the typical salt high marsh reference condition into completely different ecological sites. A brief synopsis of these external transitions and ecological sites are located near the end of the previous section on ecological dynamics.
Community 1.1
Saltmeadow cordgrass – saltgrass [saltmeadow rush] (S. patens - Disticlis spicata [Juncus gerardii)
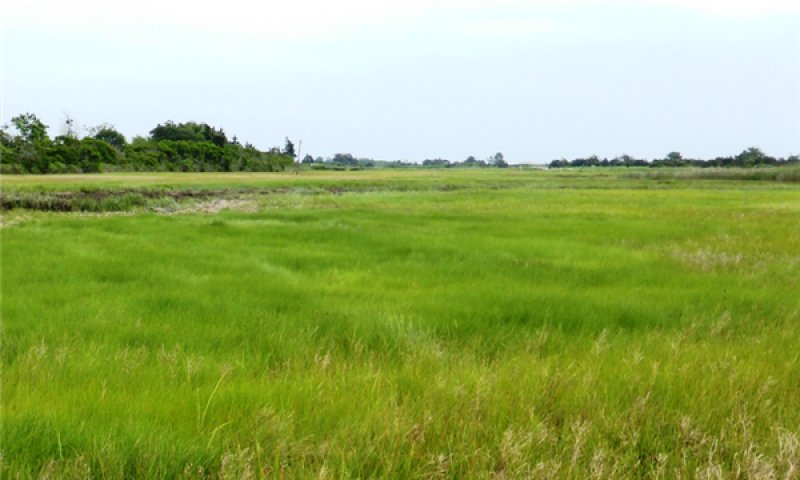
Figure 11. High marsh -Spartina patens - Distichlis spicata
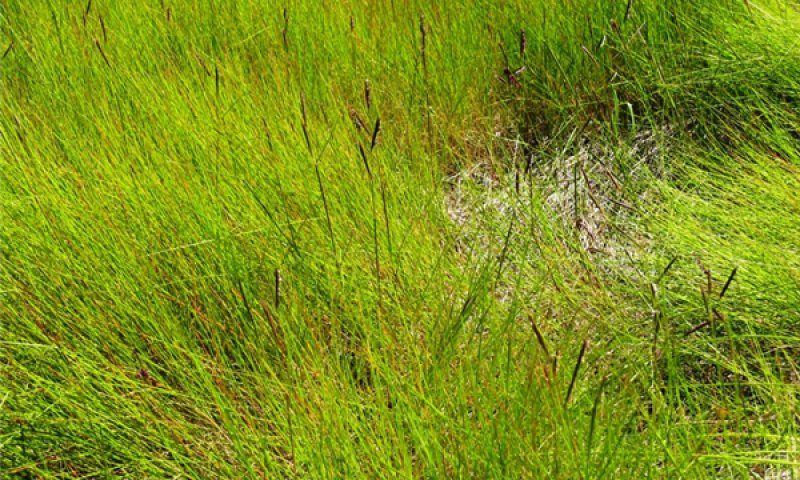
Figure 12. Spartina patens
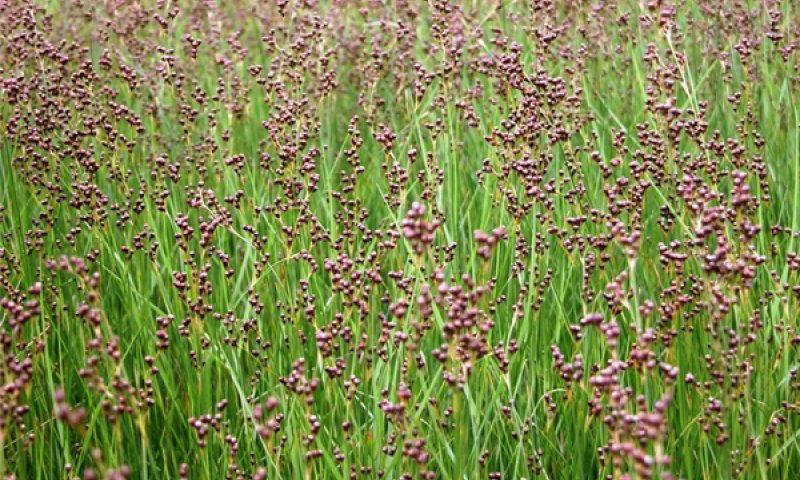
Figure 13. Juncus gerardii
The high marsh salt meadow community-type is dominated by plant species that can only withstand limited tidal submergence and duration– generally above mean high water, and thereby, flooded only periodically by spring tides that readily drain away without standing water. The predominant plants are three graminoids: Spartina patens (saltmeadow grass) and Distichlis spicata (saltgrass) and Juncus gerardii (saltmeadow rush). While these plants may grow intermixed, the high salt marsh often consists of a shifting mosaic of patches dominated by a single species. These localized patches are attributable to the different strategies of each species takes with respect to tradeoffs between stress tolerance and competition (Bertness, 1991a; Bertness and Shumway, 1993; Bertness and Hacker, 1994; Emery et al., 2001; Zhang and Shao, 2013). Spartina patens comprises the dominant plant in many large areas of salt meadow. Distichlis spicata rarely achieves the predominance of the competitive turf-forming Spartina patens or Juncus gerardii, but it is common and widespread on the high marsh. Beds of Distichlis spicata are particularly conspicuous during recovery of sites previously made bare and Distichlis spicata does occasionally participate in panne vegetation. Conversely, Juncus gerardii most commonly occurs on higher and better-drained locations of the high marsh. Other plants that exist on tidal marshes include: Solidago sempervirens (seaside goldenrod), Symphyotrichum tenuifolium (perennial saltmarsh American aster), and Symphyotrichum subulatum (eastern annual saltmarsh aster), Limonium carolinianum (lavender thrift or Carolina sea-lavender), Argentina egedii ssp. groenlandica (Pacific or coastal silverweed), and Polygonum ramosissimum ssp. ramosissimum (bushy knotweed or yellow-flowered knotweed). Species that are found much less frequently as individuals in the high marsh meadow are plants more common to the panne habitats are: Plantago maritima ssp. juncoides (goosetongue or seaside plantain), Triglochin maritima (seaside arrow grass) and Atriplex prostrata (triangle orache or hastate-leaved orache), Salicornia depressa (common glasswort or Virginia glasswort), and Spartina alterniflora (smooth cordgrass). Biomass estimates are reported from Nixon (1982).
Figure 14. Annual production by plant type (representative values) or group (midpoint values)
Table 5. Canopy structure (% cover)
Height Above Ground (ft) | Tree | Shrub/Vine | Grass/ Grasslike |
Forb |
---|---|---|---|---|
<0.5 | – | – | – | – |
>0.5 <= 1 | – | – | 50-100% | 0-1% |
>1 <= 2 | – | – | – | – |
>2 <= 4.5 | – | – | – | – |
>4.5 <= 13 | – | – | – | – |
>13 <= 40 | – | – | – | – |
>40 <= 80 | – | – | – | – |
>80 <= 120 | – | – | – | – |
>120 | – | – | – | – |
Community 1.2
Maritime marsh elder / saltmeadow rush (Iva frutescens / Juncus gerardii) upper salt marsh shrubland
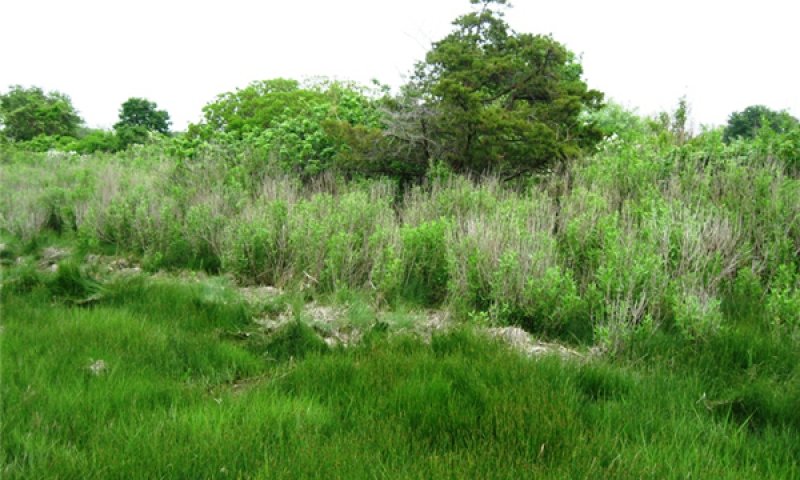
Figure 15. Upper high marsh - Iva, Juncus
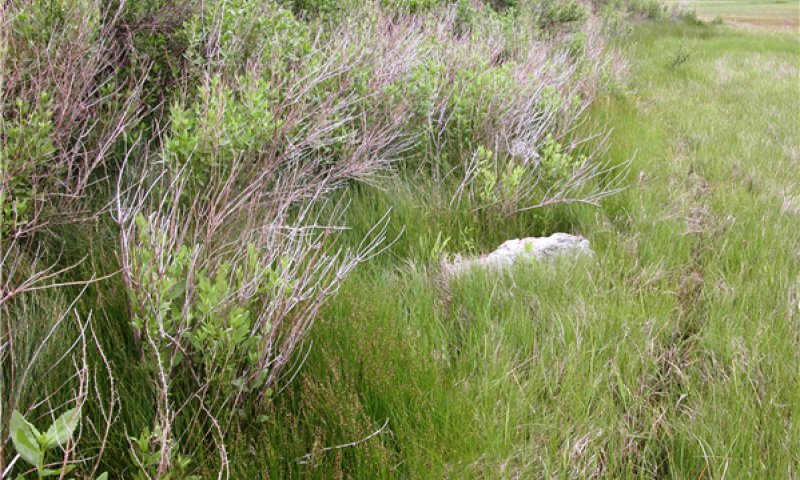
Figure 16. Juncus under Iva (Rosza)
This is an upper salt marsh shrubland community type. Under ambient marsh conditions, Iva frutescens ssp. oraria (maritime marsh elder) is rarely found on the graminoid-dominated expanses of the high marsh (Nichols, 1920; Miller and Egler, 1950; Niering and Warren, 1980; Nixon, 1982). Iva frutescens is often limited to higher elevations or the landward boundary of the salt marsh at the upland border where it may be mixed with Juncus gerardii (saltmeadow rush). Where influenced by freshwater seepage from the adjacent uplands, and brackish conditions prevail, Baccharis halimifolia var. angustior (eastern baccharis) and Panicum virgatium (switchgrass) are present with many other species more characteristic of a brackish tidal shrubland, hence treated as a separate ecological site. Iva frutescens is limited in distribution because it is somewhat salt tolerant yet intolerant of prolonged flooding (Thursby and Abdelrhman, 2004; Bertness, Gough, et al., 1992; Bertness, Wikler, et al., 1992). Furthermore, Iva frutescens is locally self-perpetuating at the upland border by facilitating its own by trapping tidally transported wrack and creating shade (Bertness and Yeh, 1994). Self-competition is reduced due to a relatively short life span 4-6 [10] years (Thursby and Abdelrhman, 2004). Biomass estimates are reported from Bertness et al. (1992).
Figure 17. Annual production by plant type (representative values) or group (midpoint values)
Table 6. Canopy structure (% cover)
Height Above Ground (ft) | Tree | Shrub/Vine | Grass/ Grasslike |
Forb |
---|---|---|---|---|
<0.5 | – | – | – | – |
>0.5 <= 1 | – | – | 0-100% | – |
>1 <= 2 | – | – | – | – |
>2 <= 4.5 | – | 0-100% | – | – |
>4.5 <= 13 | – | – | – | – |
>13 <= 40 | – | – | – | – |
>40 <= 80 | – | – | – | – |
>80 <= 120 | – | – | – | – |
>120 | – | – | – | – |
Community 1.3
Seaside arrowgrass - stunted smooth cordgrass - common glasswort [un-vegetated patches]
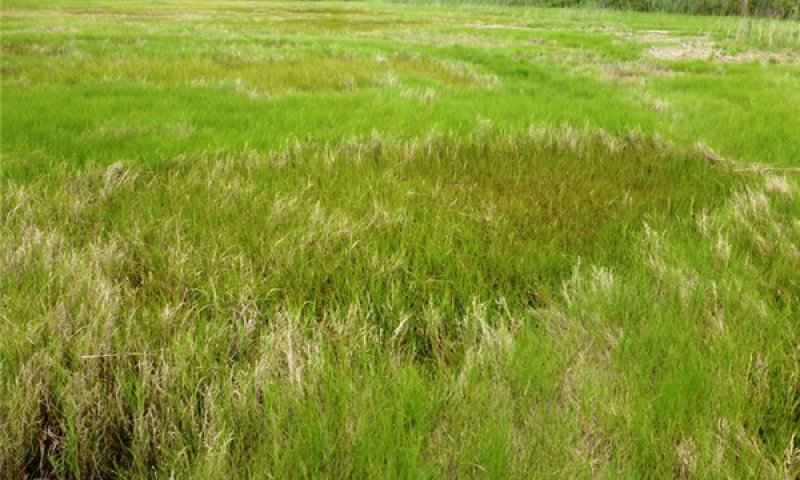
Figure 18. Stunted S.a. panne
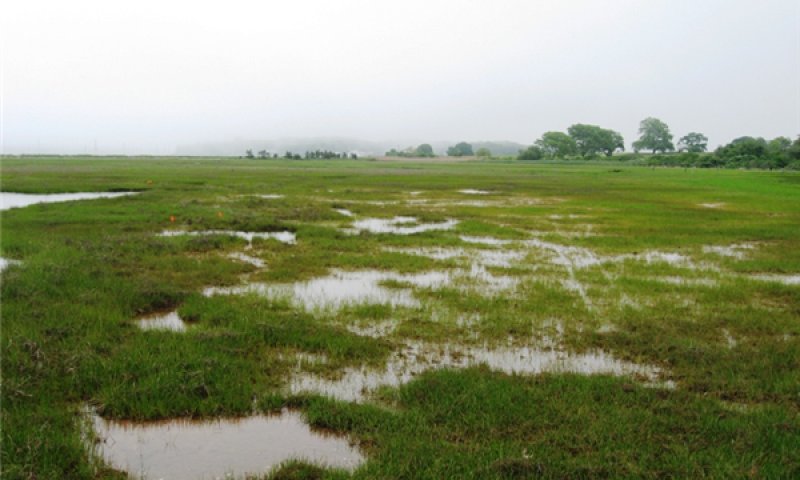
Figure 19. Flooded pannes
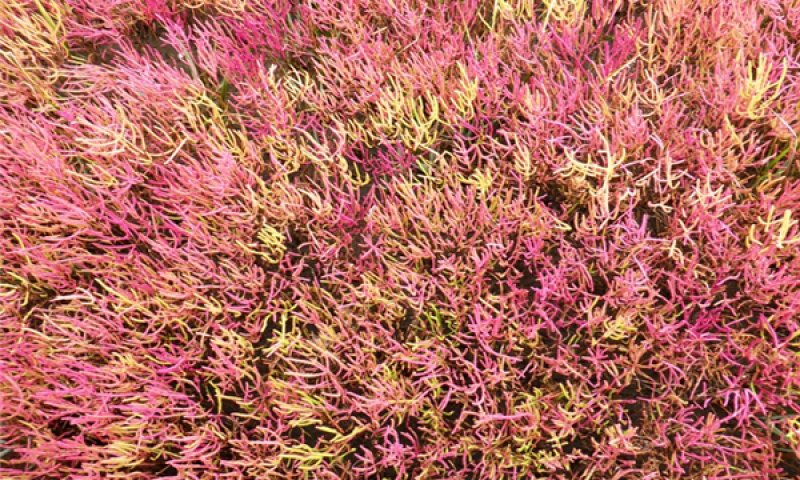
Figure 20. Salicornia pannes in Autumn
Panne vegetation of low, saturated, hypersaline depressions or flats is quite variable, hence a sequence of three panne community variants can be differentiated in the field along a gradient of increasing salinity and waterlogging (i) Triglochin maritima (seaside arrowgrass) dominated mixed forb panne, (ii) Spartina alterniflora (stunted smooth cordgrass), and (iii) un-vegetated salt panne. (i) Mixed forb pannes are typically dominated by Triglochin maritima (seaside arrow-grass). Other plants include: Plantago maritima ssp. juncoides (seaside plantain), Limonium carolinianum (Carolina sea-lavender), Atriplex prostrata (halberd-leaved orach), Agalinis maritima (saltmarsh agalinis), Argentina egedii ssp. groenlandica (coastal silverweed), and Suaeda maritima (herbaceous seepweed). (ii) Stunted Spartina alterniflora pannes possess fewer species. Spartina alterniflora (smooth cordgrass) and Salicornia depressa (common glasswort) can each be exclusively dominant or mixed. Other plant species present, but less common include: Sarcocornia perennis (woody glasswort), Atriplex prostrata (triangle orache), and Distichilis spicata (saltgrass). (iii.) Salt pannes are extreme habitats and thus, un-vegetated (Miller and Egler, 1950). Pannes and deeper pools are low, undrained depressions on the surface of the marsh, isolated from tidal creeks. Occurring primarily in the high marsh mosaic, pannes and pools, may also occasionally appear as distinctive features on the upper reaches of the low marsh. Pannes are somewhat ephemeral and only temporarily flooded, whereas pools are deeper and permanently to semi-permanently flooded. Cycles of tidal flooding and evaporation leads to hypersaline conditions (Niering and Warren, 1980; Bertness, Gough, et al., 1992) and gradients of salinity exceeding 40 to 50 ppt (62.5 – 78.1 dSm-1) and standing water depth and duration correlate to a sequence of vegetative cover and composition (Miller and Egler, 1950). The lowest part of the panne, which tends to be more waterlogged and more saline, may be un-vegetated. As waterlogging and salinity diminish with increase elevation, Salicornia spp. and stunted Spartina alterniflora grow, followed next by a mixed and highly variable assemblage of forbs. These three communities may exist in layers of concentric rings determined by microrelief or as separate pannes outright. Although floristically distinctive, the vegetation cover and composition of pannes are also quite variable and can change from year to year (Miller and Egler, 1950). For these reasons, and despite these variations, all three community-types: un-vegetated salt pannes, stunted Spartina alterniflora pannes, and mixed forb pannes will be treated together as variants of the saltmarsh panne. Biomass estimates are reported from Tiner (2013).
Figure 21. Annual production by plant type (representative values) or group (midpoint values)
Table 7. Annual production by plant type
Plant type | Low (lb/acre) |
Representative value (lb/acre) |
High (lb/acre) |
---|---|---|---|
Forb | 2200 | 4100 | 6200 |
Total | 2200 | 4100 | 6200 |
Table 8. Canopy structure (% cover)
Height Above Ground (ft) | Tree | Shrub/Vine | Grass/ Grasslike |
Forb |
---|---|---|---|---|
<0.5 | – | – | 0-85% | – |
>0.5 <= 1 | – | – | – | – |
>1 <= 2 | – | – | – | – |
>2 <= 4.5 | – | – | – | – |
>4.5 <= 13 | – | – | – | – |
>13 <= 40 | – | – | – | – |
>40 <= 80 | – | – | – | – |
>80 <= 120 | – | – | – | – |
>120 | – | – | – | – |
Community 1.4
Widgeongrass or beaked ditch-grass (Ruppia maritima) marsh pools
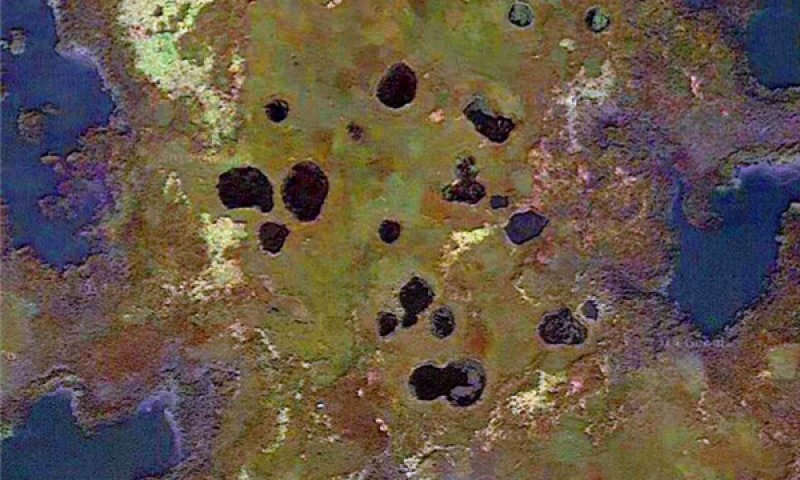
Figure 22. Stratford Great Meadow pools (aerial)
Pools on the high salt marsh are soft bottom depressions that are permanently to semi permanently flooded. Pools do not dry out as shallower pannes do (Harshberger, 1916; Miller and Egler, 1950; Redfield, 1972). These deepwater pools provide habitat for the submerged aquatic Ruppia maritima (widgeongrass or beaked ditch-grass) often with marine algae. In brackish conditions Ruppia maritima may overlap with other aquatics: Zannichellia palustris (horned pondweed) and Stuckenia pectinata (sago pondweed). Based on an area-wide survey throughout coastal New England (Adamowicz and Roman, 2005), pools on average were 200 m2 at densities estimated at 13 pools/ha. Historically, pannes and pools were more common features on the high marsh. The scarcity of salt marsh pools today is a legacy of intensive ditching for mosquito control and salt hay operations (Ewanchuk and Bertness, 2004; Adamowicz and Roman, 2005).
Pathway 1.1A
Community 1.1 to 1.2
![Saltmeadow cordgrass – saltgrass [saltmeadow rush] (S. patens - Disticlis spicata [Juncus gerardii)](https://edit.jornada.nmsu.edu/uploads/med/es/pc/7269.jpg)
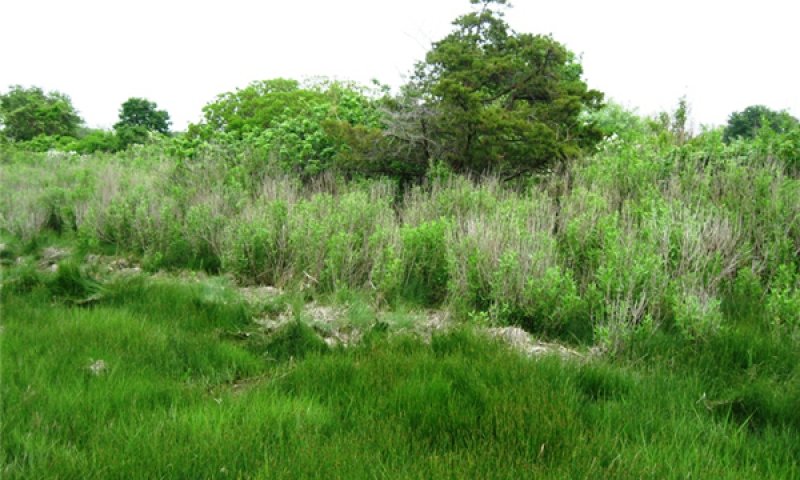
Marsh accretion coupled with wrack increases marsh elevation to create conditions favorable for conversion to Iva frutescens / Juncus gerardii shrub land.
Pathway 1.1B
Community 1.1 to 1.3
![Saltmeadow cordgrass – saltgrass [saltmeadow rush] (S. patens - Disticlis spicata [Juncus gerardii)](https://edit.jornada.nmsu.edu/uploads/med/es/pc/7269.jpg)
![Seaside arrowgrass - stunted smooth cordgrass - common glasswort [un-vegetated patches]](https://edit.jornada.nmsu.edu/uploads/med/es/pc/7291.jpg)
Agents of natural disturbance such as wrack, ice-scouring, or lack of accretion, that erode, compact, subside, or otherwise diminish the marsh elevation and create greater stress through waterlogging and elevated salinity, create panne habitat and favor stress-tolerant species such as Spartina alterniflora and forbs.
Pathway 1.1C
Community 1.1 to 1.4
![Saltmeadow cordgrass – saltgrass [saltmeadow rush] (S. patens - Disticlis spicata [Juncus gerardii)](https://edit.jornada.nmsu.edu/uploads/med/es/pc/7269.jpg)
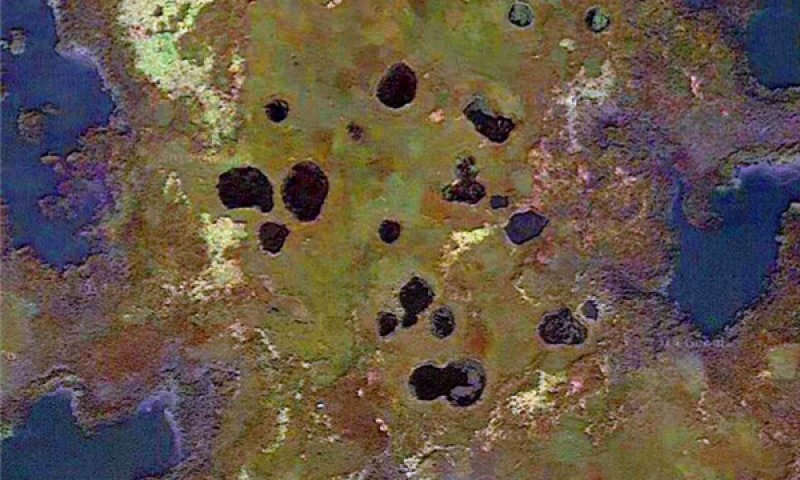
Agents of natural disturbance that allow for permanent standing water create Ruppia marsh pools including wrack, ice-scouring, or lack of accretion.
Pathway 1.2A
Community 1.2 to 1.1
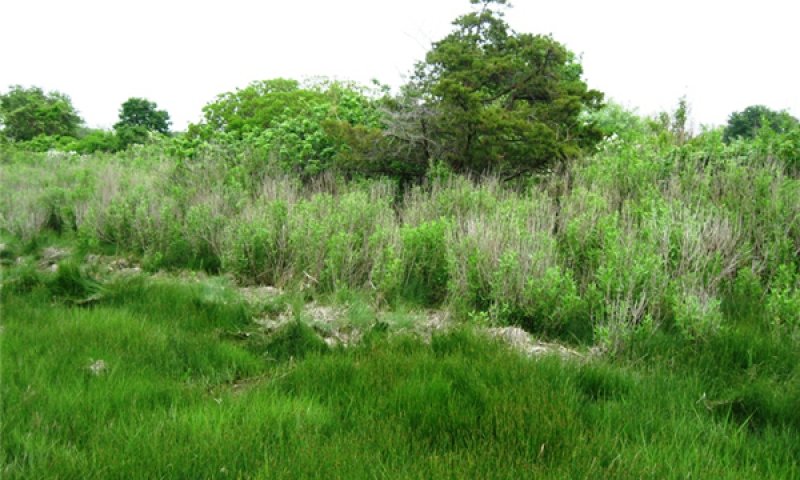
![Saltmeadow cordgrass – saltgrass [saltmeadow rush] (S. patens - Disticlis spicata [Juncus gerardii)](https://edit.jornada.nmsu.edu/uploads/med/es/pc/7269.jpg)
Marsh erosion, subsidence, or compaction that result from ice-scouring, or lack of accretion, can diminish elevation or increase waterlogging that can disfavor and transform Iva frutescens and favor the high marsh mosaic.
Pathway 1.2B
Community 1.2 to 1.3
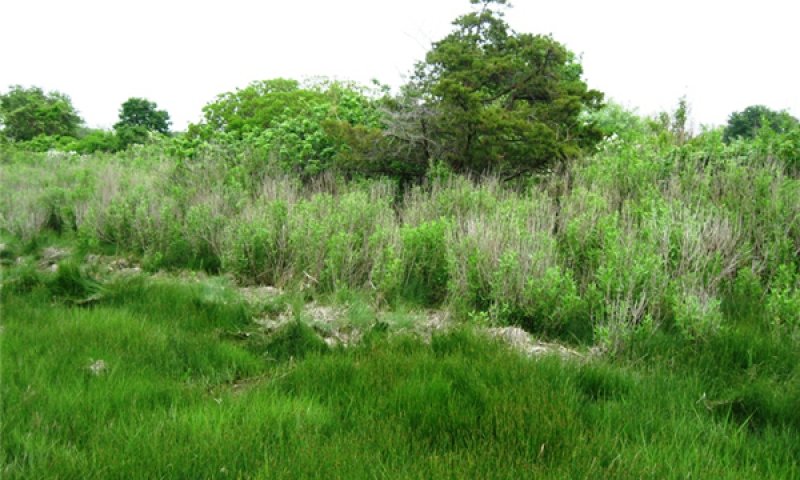
![Seaside arrowgrass - stunted smooth cordgrass - common glasswort [un-vegetated patches]](https://edit.jornada.nmsu.edu/uploads/med/es/pc/7291.jpg)
Agents of natural disturbance such as wrack, ice-scouring, or lack of accretion, that erode, compact, subside, or otherwise that diminish the marsh elevation and create greater stress through waterlogging and elevated salinity, create panne habitat and favor stress-tolerant species such as Spartina alterniflora and forbs.
Pathway 1.2C
Community 1.2 to 1.3
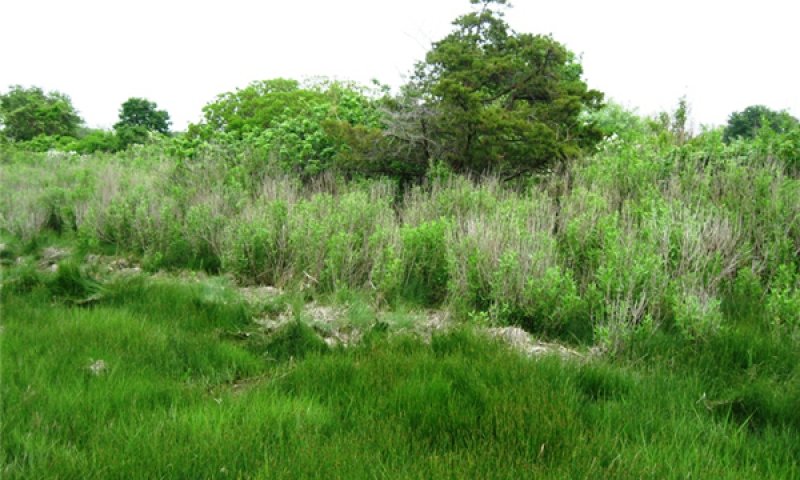
![Seaside arrowgrass - stunted smooth cordgrass - common glasswort [un-vegetated patches]](https://edit.jornada.nmsu.edu/uploads/med/es/pc/7291.jpg)
Agents of natural disturbance that allow for permanent standing water create marsh pools with Ruppia maritima (beaked ditch-grass).
Pathway 1.3A
Community 1.3 to 1.1
![Seaside arrowgrass - stunted smooth cordgrass - common glasswort [un-vegetated patches]](https://edit.jornada.nmsu.edu/uploads/med/es/pc/7291.jpg)
![Saltmeadow cordgrass – saltgrass [saltmeadow rush] (S. patens - Disticlis spicata [Juncus gerardii)](https://edit.jornada.nmsu.edu/uploads/med/es/pc/7269.jpg)
Marsh accretion and facilitation by panne forbs to lessen waterlogging and salinity stress allow panne species to succeed to high marsh meadow.
Pathway 1.3B
Community 1.3 to 1.1
![Seaside arrowgrass - stunted smooth cordgrass - common glasswort [un-vegetated patches]](https://edit.jornada.nmsu.edu/uploads/med/es/pc/7291.jpg)
![Saltmeadow cordgrass – saltgrass [saltmeadow rush] (S. patens - Disticlis spicata [Juncus gerardii)](https://edit.jornada.nmsu.edu/uploads/med/es/pc/7269.jpg)
Significant marsh accretion coupled with wrack effects increase marsh elevation and create conditions favorable for conversion to Iva frutescens / Juncus gerardii shrub land. (This is not common.)
Pathway 1.3C
Community 1.3 to 1.4
![Seaside arrowgrass - stunted smooth cordgrass - common glasswort [un-vegetated patches]](https://edit.jornada.nmsu.edu/uploads/med/es/pc/7291.jpg)
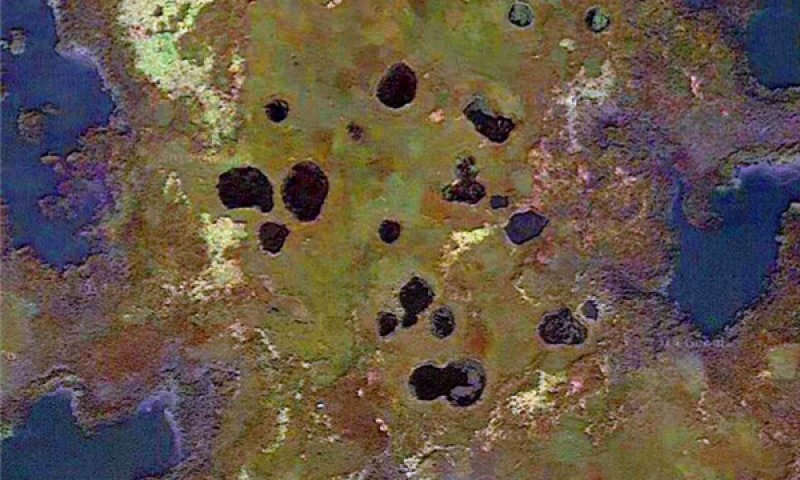
Agents of natural disturbance allow for permanent standing water create marsh pools of Ruppia (beaked ditch-grass).
Pathway 1.4A
Community 1.4 to 1.3
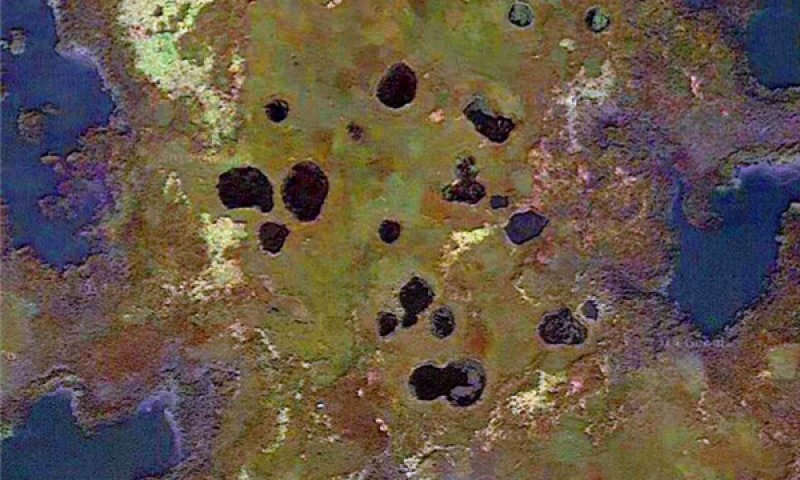
![Seaside arrowgrass - stunted smooth cordgrass - common glasswort [un-vegetated patches]](https://edit.jornada.nmsu.edu/uploads/med/es/pc/7291.jpg)
Natural disturbances eliminate the permanent nature of the pool, e.g., wrack or depositional sediment that act as fill or erosion or peat collapse that transforms the pool to an intermittently-drained panne with a wide mix of possible replacement plants of varying stress tolerance ranging from Spartina alterniflora (smooth cordgrass) and Salicornia spp. (glassworts) to various forb mixes, such as Triglochin maritima (seaside arrow-grass) and Plantago maritima (seaside plantain).
State 2
Hydrologically-altered Marsh
Human disturbances in salt marshes such as ditching and ditching legacy, accelerated sea-level rise, and open water marsh management (OMWM) have altered hydrologic conditions in many ways which in turn affect the vegetation. Hydrologically-altered habitat includes both, drier and wetter scenarios. In the drier situation occur local levees and ditch margins of (2.1) Iva frutescens (maritime marsh-elder). In the wetter situations occur either flooded drainage ditch banks of (2.2) Spartina alterniflora (smooth cordgrass), and or excavated OMWM pools (2.3) Ruppia maritima (beaked ditch-grass) and marine algae, or various degrees of waterlogging in un-drained pannes with (2.4) Distichilis spicata (salt grass)- Salicornia spp. (glassworts) - Spartina alterniflora (smooth cordgrass) or un-vegetated.
Community 2.1
Maritime marsh elder (Iva frutescens ssp. oraria) levees
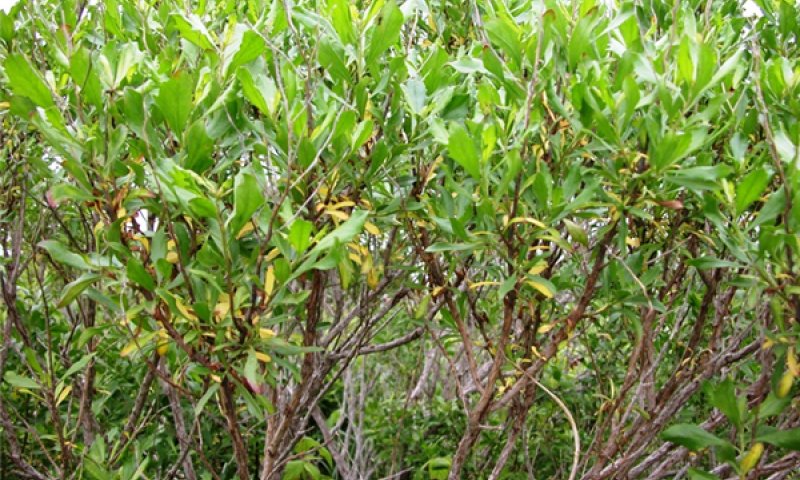
Figure 23. Iva frutescens
Iva frutescens ssp. oraria (maritime marsh elder) vegetation-type can be indicative of disturbed sites on the high marshes, where extensive ditching has allowed for greater drainage, or higher elevations such as normal depositional levees or artificial levees of side-cast ditch spoils (Bourn and Cottam 1950, Miller and Egler 1950, Niering and Warren 1980). Biomass estimates reported from Bertness et al. (1992).
Figure 24. Annual production by plant type (representative values) or group (midpoint values)
Table 9. Canopy structure (% cover)
Height Above Ground (ft) | Tree | Shrub/Vine | Grass/ Grasslike |
Forb |
---|---|---|---|---|
<0.5 | – | – | – | – |
>0.5 <= 1 | – | – | 0-100% | – |
>1 <= 2 | – | – | – | – |
>2 <= 4.5 | – | 0-75% | – | – |
>4.5 <= 13 | – | – | – | – |
>13 <= 40 | – | – | – | – |
>40 <= 80 | – | – | – | – |
>80 <= 120 | – | – | – | – |
>120 | – | – | – | – |
Community 2.2
Smooth cordgrass [intermediate to tall] (Spartina alterniflora [intermediate to tall]) ditches
Spartina alterniflora (smooth cordgrass) of the intermediate to tall form proliferates along the ditch-banks (Nichols 1920, Miller and Egler 1950, Niering and Warren 1980, Bertness 1992). Parallel ditching has created low-marsh habitat amenable to Spartina alterniflora, comparable to natural creek-bank habitats. If ditches lack the tidal-flow to keep them scoured, they eventually aggrade and/or slump and enlarge into long narrow low marshes made conspicuous by the proliferation of Spartina alterniflora (Bourn and Cottam 1950, Miller and Egler 1950). Biomass estimates are reported from Tiner (2013).
Figure 25. Annual production by plant type (representative values) or group (midpoint values)
Table 10. Canopy structure (% cover)
Height Above Ground (ft) | Tree | Shrub/Vine | Grass/ Grasslike |
Forb |
---|---|---|---|---|
<0.5 | – | – | – | – |
>0.5 <= 1 | – | – | – | – |
>1 <= 2 | – | – | 0-100% | – |
>2 <= 4.5 | – | – | – | – |
>4.5 <= 13 | – | – | – | – |
>13 <= 40 | – | – | – | – |
>40 <= 80 | – | – | – | – |
>80 <= 120 | – | – | – | – |
>120 | – | – | – | – |
Community 2.3
Widgeongrass or beaked ditch-grass (Ruppia maritima) excavated ponds
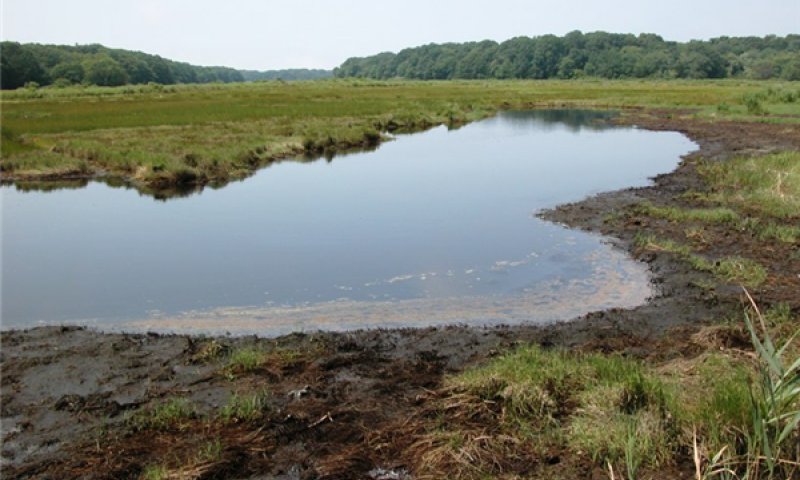
Figure 26. Open Marsh Water Mmgt created pool
Many marsh restoration projects, open marsh water management, and integrated marsh management, involve re-establishing or creating permanently flooded pools to retain the larvivorous fish, e.g., Fundulus hereroclitus (mummichog) and Cyprinodon variegatus (sheepshead minnow) for mosquito control (James-Pirri et al. 2012, Rochlin et al. 2012b) and larger ponds to provide resting and foraging habitat of Ruppia maritima (beaked ditch-grass) for waterfowl (Erwin et al. 1994).
Community 2.4
Saltgrass - glasswort - smooth cordgrass - [unvegetated patches] (Distichlis spicata - Salicornia sp
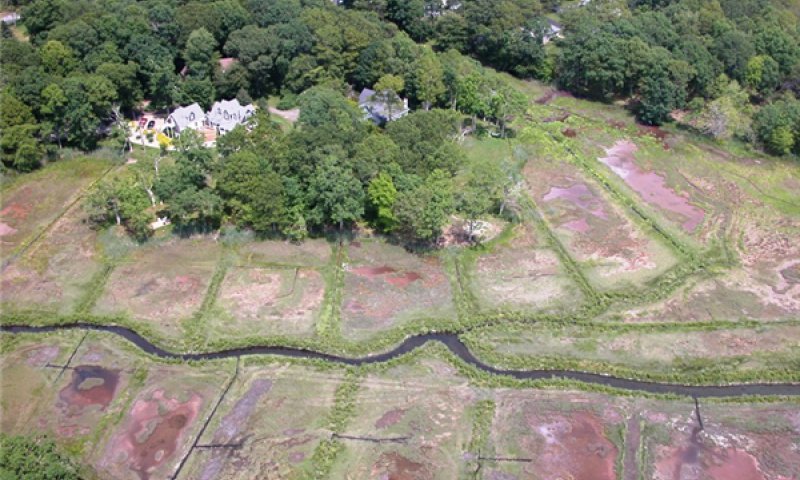
Figure 27. Ditch "panne" panels between creeks
Secondary panne formation commonly occur in disturbed or waterlogged sites that are legacies of past ditching, open marsh water management (OMWM) practices, and excessive nutrient enrichment (Warren and Niering, 1993; Adamowicz and Roman, 2002). Distinguishing between altered sites and reference sites, both characterized by similar species is possible by knowledge of local history. Ironically, ditches, the main activity used to drain marshes and eliminate pannes and pools, can over time also lead to wetter marshes (Miller and Egler, 1950; Teal, 1986; Warren and Niering, 1993). Tidal ditches, like tidal creeks, form levees and associated waterlogged inter-ditch pannes which flood more frequently than an un-fragmented high marsh. Side-cast ditch spoils also create artificial levees. Waterlogging is also associated with various practices of OMWM, such as ditch plugging, runnelling (shallow ditches) and pool excavation. Also ironically, nutrient enrichment that results in greater aboveground growth actually reduces the accumulation of belowground biomass and stability causing marsh subsidence (Turner et al., 2009; Deegan et al., 2012) which can lead to panne formation and waterlogging. Akin to natural pannes, these disturbed secondary pannes that are highly waterlogged are dominated by Spartina alterniflora (smooth cordgrass) and Salicornia spp. (glassworts) or occasionally un-vegetated, sometimes with a mat of cyanobacteria or filamentous green alga, Cladophora gracilis. Less commonly are secondary disturbed pannes with Distichlis spicata (saltgrass) and/or mixed forbs.
Pathway 2.1A
Community 2.1 to 2.4
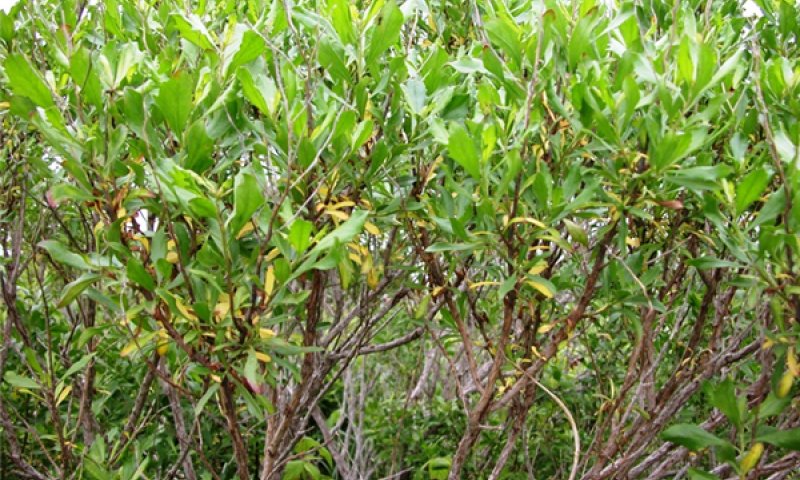
![Saltgrass - glasswort - smooth cordgrass - [unvegetated patches] (Distichlis spicata - Salicornia sp](https://edit.jornada.nmsu.edu/uploads/med/es/pc/7299.jpg)
Over time as ditches aggrade and fail, the levees eventually collapse. Further marsh manipulations such as open water marsh management, OMWM, and ditch plugging may hasten waterlogging and create disturbed secondary pannes. These areas support typical panne forbs and Spartina alterniflora (smooth cordgrass) and Dstichilis spicata (saltgrass). This process may be hastened by eutrophication.
Conservation practices
Wetland Wildlife Habitat Management |
---|
Pathway 2.2A
Community 2.2 to 2.4
Over time as ditches aggrade and fail, waterlogged marsh and secondary pannes form. Eutrophication may hasten this process. Further marsh manipulations such as open marsh water management and ditch plugging may also hasten waterlogging and panne formation.
Conservation practices
Wetland Wildlife Habitat Management |
---|
Pathway 2.3A
Community 2.3 to 2.4
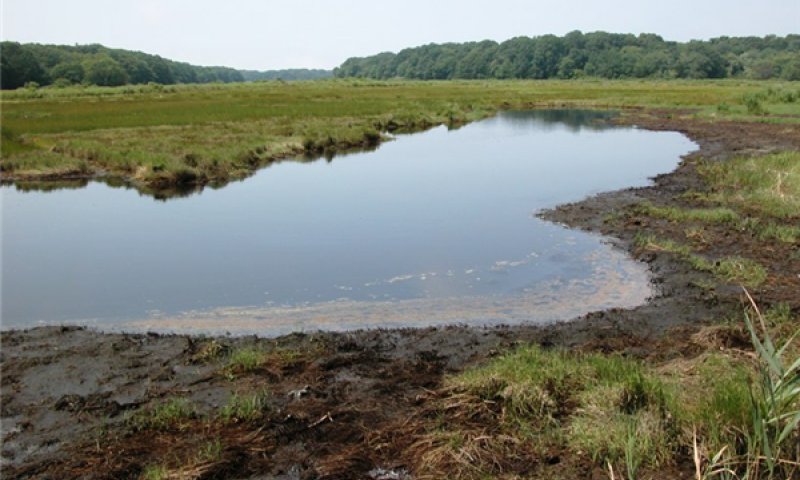
![Saltgrass - glasswort - smooth cordgrass - [unvegetated patches] (Distichlis spicata - Salicornia sp](https://edit.jornada.nmsu.edu/uploads/med/es/pc/7299.jpg)
Created or natural marsh pools may (but rarely) fail leading to waterlogging and pannes formation. Further marsh manipulations such as open marsh water managment and ditch plugging may hasten waterlogging and panne formation.
Conservation practices
Wetland Wildlife Habitat Management |
---|
Pathway 2.4A
Community 2.4 to 2.2
Ditching and runnelling effectively creates low marsh habitat along ditch banks favorable to Spartina alternifora (smoothcordgrass).
Conservation practices
Wetland Wildlife Habitat Management | |
---|---|
Wetland Enhancement |
Pathway 2.4B
Community 2.4 to 2.3
![Saltgrass - glasswort - smooth cordgrass - [unvegetated patches] (Distichlis spicata - Salicornia sp](https://edit.jornada.nmsu.edu/uploads/med/es/pc/7299.jpg)
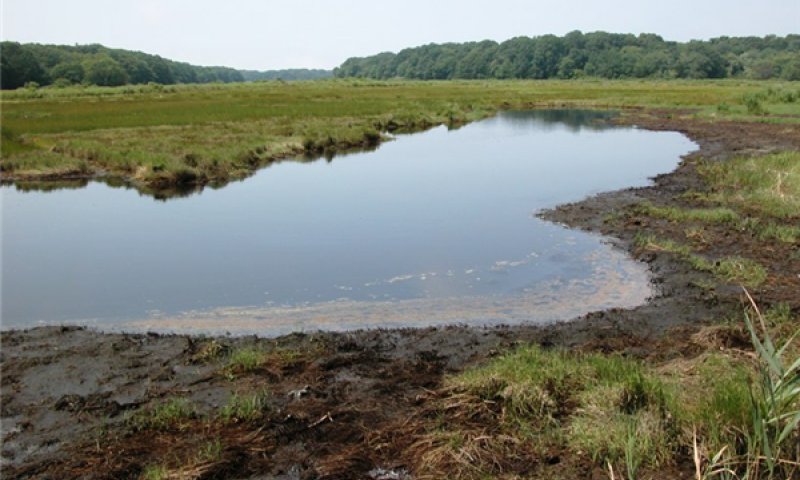
Artificial marsh pools and ponds are created by surface excavations designed to not drain away and permanently hold water.
Conservation practices
Wetland Wildlife Habitat Management | |
---|---|
Wetland Enhancement |
State 3
Tidally-restricted State
Marsh surface modifications and engineered structures, e.g., filled causeways, constricted bridges, undersized and improperly emplaced culverts, cause tidal restrictions (Roman, 2012; Tiner, 2013). Tidal restrictions reduce tidal flooding, lower salinity, and may lower the water table, changing the character of the salt marsh which prompts a state change in the vegetation. The type of replacement vegetation depends upon the nature of the restriction and the local drainage pattern. Open restrictions, such as culverts, are common and have less impact then closed restrictions that prohibit tidal exchange entirely (Crain et al., 2009).
Community 3.1
Common reed - cattails (Phragmites australis - Typha spp.) tidally-restricted marsh
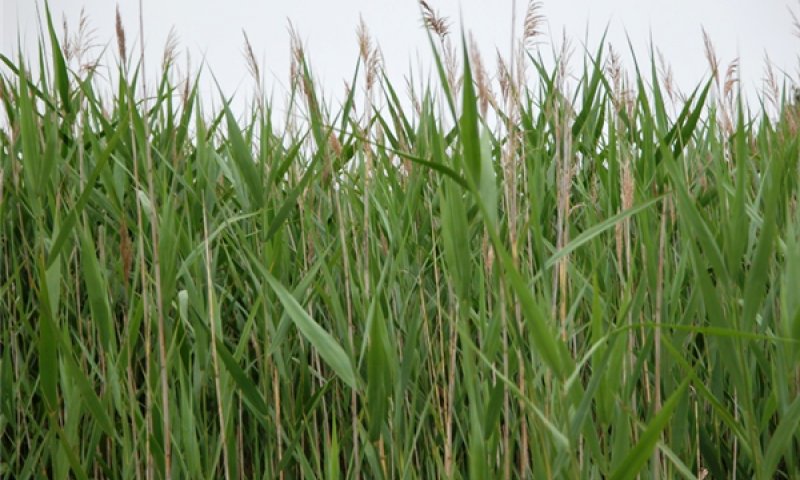
Figure 28. Non-native Phragmites australis
Due to tidal restrictions, reduced salinities in the range of 0.5 to 18 ppt (0.8 – 28.1 dSm-1) result in in the conversion of salt marsh species to more competitive brackish marsh species, such as Typha angustifolia (narrow-leaved cattail) and Typha x glauca (hybrid cattail), and Schoenoplectus spp. (bulrushes) as well as invasive Phragmites australis (common reed), or even possibly the native genotype, Phragmites australis ssp. americanus (American reed). The vegetation is typically a dense mosaic often dominated by a single species with scattered occurrences of other common brackish species, like Hibiscus moscheutos (crimson-eyed rose mallow), Pluchea odorata var. succulenta (sweetscent), Mikania scandens (climbing hempvine), and Solidago sempervirens (seaside goldenrod). Eutrophication may favor the establishment of invasive Phragmites (Amsberry et al. 2000).
Figure 29. Annual production by plant type (representative values) or group (midpoint values)
Table 11. Canopy structure (% cover)
Height Above Ground (ft) | Tree | Shrub/Vine | Grass/ Grasslike |
Forb |
---|---|---|---|---|
<0.5 | – | – | – | – |
>0.5 <= 1 | – | – | – | – |
>1 <= 2 | – | – | 0-100% | – |
>2 <= 4.5 | – | – | – | – |
>4.5 <= 13 | – | – | – | – |
>13 <= 40 | – | – | – | – |
>40 <= 80 | – | – | – | – |
>80 <= 120 | – | – | – | – |
>120 | – | – | – | – |
Transition T1A
State 1 to 2
Physical alterations to marsh hydrology by cut and fill, ditching, open water marsh management can add or subtract tidal flooding, affecting shifts and changes among salt plant communities. Replacement plant communities are variable and include Iva frutenscens (maritime marsh elder) ditch levees/margins, Spartina alterniflora (smooth cordgrass) ditch banks, and excavated pools with Ruppia (beaked ditch-grass) and marine algae.
Transition T1B
State 1 to 3
Engineered structures, such as culverts, tide gates, etc., that restrict the tidal flooding of the marsh will ultimately change the vegetation to a brackish to freshwater state, often supporting the invasive Phragmites (common reed). In extreme cases, the site may be rendered unvegetated.
Restoration pathway R2A
State 2 to 1
Restoration of salt high marsh requires correcting the hydrological modifications at issue, such as selective filling of ditches or excavations and or compensating for the elevation changes that lead to waterlogging. To address waterlogging concerns, Runnelling (shallow ditches) is still considered an experimental treatment in these situations.
Transition T2A
State 2 to 3
Engineered structures, such as culverts, tide gates, etc., which restrict the tidal flooding of the marsh will ultimately change the vegetation to a brackish to freshwater state, often supporting the invasive Phragmites (common reed). In extreme cases, the site may be rendered unvegetated.
Restoration pathway R3A
State 3 to 1
Restoration involves removing or fixing the tidal restrictions (e.g., culvert size, location, invert, etc.) and reintroducing tidal flooding. Regulating the tides or compensating for surface elevation loss from subsidence using fill or engineering can facilitate the conversion back to salt high marsh. If elevation grades are lower salt low marsh restoration is possible.
Conservation practices
Wetland Wildlife Habitat Management | |
---|---|
Wetland Restoration | |
Wetland Enhancement |
Transition T3A
State 3 to 2
Removing or fixing the tidal restrictions (e.g., culvert size, location, invert, etc.) with engineering or land management and renewing the ditches to facilitate drainage. Although the resulting marsh may be hydrologically-altering, the return of salt marsh plants may be considered a partial restoration.
Additional community tables
Table 12. Community 1.1 plant community composition
Group | Common name | Symbol | Scientific name | Annual production (lb/acre) | Foliar cover (%) | |
---|---|---|---|---|---|---|
Grass/Grasslike
|
||||||
1 | salt high meadow | 2500–10000 | ||||
saltmeadow cordgrass | SPPA | Spartina patens | 2676–9814 | 50–100 | ||
saltgrass | DISP | Distichlis spicata | 3211–8833 | 50–100 | ||
saltmeadow rush | JUGE | Juncus gerardii | 3792–5085 | 50–100 |
Table 13. Community 1.2 plant community composition
Group | Common name | Symbol | Scientific name | Annual production (lb/acre) | Foliar cover (%) | |
---|---|---|---|---|---|---|
Shrub/Vine
|
||||||
1 | Iva-Juncus upper marsh | 0 | ||||
Jesuit's bark | IVFRO2 | Iva frutescens ssp. oraria | 0–2500 | 0–100 | ||
Grass/Grasslike
|
||||||
2 | Iva-Juncus upper marsh | 0 | ||||
saltmeadow rush | JUGE | Juncus gerardii | 0 | 0–100 |
Table 14. Community 1.3 plant community composition
Group | Common name | Symbol | Scientific name | Annual production (lb/acre) | Foliar cover (%) | |
---|---|---|---|---|---|---|
Grass/Grasslike
|
||||||
1 | high marsh panne | 2200–6200 | ||||
smooth cordgrass | SPAL | Spartina alterniflora | 2200–6200 | 0–85 | ||
Forb
|
||||||
2 | salt marsh panne | 0–2100 | ||||
Virginia glasswort | SADE10 | Salicornia depressa | 0–2100 | 0–75 | ||
seaside arrowgrass | TRMA20 | Triglochin maritima | 0 | 0–50 | ||
lavender thrift | LICA17 | Limonium carolinianum | 0 | 0–5 |
Table 15. Community 2.1 plant community composition
Group | Common name | Symbol | Scientific name | Annual production (lb/acre) | Foliar cover (%) | |
---|---|---|---|---|---|---|
Shrub/Vine
|
||||||
3 | Iva frutescens ditch margin | 0–1900 | ||||
Jesuit's bark | IVFRO2 | Iva frutescens ssp. oraria | 0–1900 | 0–75 |
Table 16. Community 2.2 plant community composition
Group | Common name | Symbol | Scientific name | Annual production (lb/acre) | Foliar cover (%) | |
---|---|---|---|---|---|---|
Grass/Grasslike
|
||||||
1 | Spartina alterniflora ditch | 3800–12000 | ||||
smooth cordgrass | SPAL | Spartina alterniflora | 3800–12000 | 0–100 |
Table 17. Community 3.1 plant community composition
Group | Common name | Symbol | Scientific name | Annual production (lb/acre) | Foliar cover (%) | |
---|---|---|---|---|---|---|
Grass/Grasslike
|
||||||
1 | reed marsh | 5100–24000 | ||||
European common reed | PHAUA7 | Phragmites australis ssp. australis | 0–25000 | 0–100 | ||
narrowleaf cattail | TYAN | Typha angustifolia | 0–12000 | 0–100 |
Interpretations
Animal community
Wildlife Habitat General Information
Wildlife use the salt tidal marsh in a variety of ways as either resident or migratory species. The most conspicuous are the macrofauna, which include the many invertebrates, including insects and spiders, and molluscs, crabs and snails, as well as fish, reptiles, birds, and mammals.
Invertebrate Habitat
Since the tidal environment is alternately flooded and exposed, tidal marshes provide habitat conditions that range from somewhat terrestrial to suitably aquatic (Tiner 2013). Terrestrial invertebrates are dominated by insects and spiders that thrive in the high marsh and access the low marsh at low tides. Whereas, marine invertebrates that dominate the low marsh, intertidal and subtidal flats are characterized by and assortment of snails, crabs, molluscs and worms. Swimming invertebrates such as shrimp (Palaemonetes) move in and out with the tides.
There are many kinds of insects and spiders found in the salt marsh, mostly confined to higher elevations (Teal 1986). The most conspicuous insects are, of course, are the biting insects including the salt marsh mosquitos (Ochlerotatus [formerly Aedes] sollicitans, O. cantator), greenheads (Tabanus nigrovittatus), and no-see-ums (Culicoides spp.). Insect herbivores include chewers and suckers. The predominant chewing insect are grasshoppers (Conocephalus spp.), katydids (Orchelimum spp.) and thrips (Anaphothrips spp.). Sucking insects are much more abundant. The predominant sucking insect is the planthopper (Prokelisia marginata). Others include plant bugs (Miridae, Trigontylus), aphids (Aphidae), scale insects (Coccoidea) and fly larvae of many species (e.g., Chloropids, Dolichopodids, and Ephydrids). Insect predators include many spiders like web-weavers (Grammonota inornata and Dictyna roscida), sac spider (Clubiona maritima), and the wolf spider (Pardosa distincta). Additional information may be found in Tiner (2013), Teal (1986), and Nixon (1982).
Typically, snail species more are common to the high marsh whereas most crab species inhabit the low marsh. Common snails include the common periwinkle (Littorina littorea) and the rough periwinkle (Littorina saxatilis) and the pulmonate salt marsh snail (Melampus bidentatus). These snail feed by scraping off algae and detritus from tidal marsh surfaces (Teal 1986). The salt marsh snail is often seen climbing the stalks of Spartina alterniflora during the flood tide to avoid inundation and predation from fishes and crabs (Tiner 2013).
Burrowing crabs leave conspicuous holes at the edges of creeks and ditches. The common fiddler crab (Uca pugnax) prefers burrowing and deposit-feeding in the stable peaty muck of the low marsh which potentially aerates the marsh soils at ebb tide and boosts the growth and productivity of Spartina alterniflora (Bertness 1985). The native herbivorious purple marsh crab (Sesarma reticulatum) has been implicated in low marsh dieoff, due to overgrazing pressures in some marshes where the populations were not held in check by predatory fish that were removed by overfishing(Holdredge et al. 2009, Altieri et al. 2012, Coverdale et al. 2013a). Ironically, the predatory nonnative European green grab, Carcinus maenas, may keep the populations of the purple marsh crab in check and reverse the effects over-grazing (Bertness and Coverdale 2013).
Fish Habitat
Tidal marshes provide sheltered shallow water habitat, nursery and foraging habitat for numerous fishes (Teal 1986, Tiner 2013). Several small fishes that spend most of their lives within high marsh pools and in creeks during the advancing tides are : mummichog (Fundulus heteroclitus), Sheepshead minnow (Cyprinodon variegatus), Atlantic silverside (Menidia menidia), Striped killifish (Fundulus majalis), Four-spined stickleback (Apeltes quadracus), and Three-spined stickleback (Gasterosteus aculeatus). Common fishes that use the tidal marsh mainly as a nursery area are: Winter flounder (Pseudopleuronectes americanus), Tautog (Tautoga onitis), Sea bass (Centropristes striata), Alewife (Alosa pseudoharengus), Menhaden (Brevoortia tyrannus), Bluefish (Pomatomus saltatrix), and Mullet (Mugil cephalus). Additional species may be found in Tiner (2013).
Reptile Habitat
Excepting sea-turtles, which are essentially marine, the diamondback terrapin (Malaclemys terrapin) commonly feeds in the high marsh ditches and creeks and low marsh. Terrapins have declined due to coastal development and collecting pressures (Teal 1986).
Bird Habitat
One of the most widely recognized values of salt marshes is their support of habitat for birds. Tidal wetlands provide a year-round home for resident birds and serve as important stopover areas for breeding, feeding and overwintering of migratory birds along the Atlantic flyway. (Teal 1986, Tiner 2013). Those birds in-and-around tidal marshes include (but not limited to), the marsh specialists that live and feed in the salt marshes: Willet (Catoptrophorus semipalmatus), Clapper Rail (Rallus longirostris), Saltmarsh sharp-tailed sparrow (Ammodramus caudacutus), Seaside sparrow (Ammodramus maritimus); waders that stalk small fishes and crustaceans: Great blue heron (Ardea herodias), Glossy ibis (Plegadis falcinellus), Great egret (Ardea alba), Snowy egret (Egretta thula), shorebirds probe for invertebrates: Killdeer (Charadrius vociferus), Greater yellowlegs (Tringa melanoleuca), Lesser yellowlegs (Tringa flavipes), Spotted sandpiper (Actitis macularia), and other sandpipers of the Calidrid tribe (Calidris spp.); seabirds that may feed in open water on the marsh: gulls (Laridae), terns (Sternidae); waterbirds: Double-crested cormorant (Phalacrocorax auritus); waterfowl: Mute swan (Cygnus olor), Canada goose (Branta canadensis) that graze on Spartina alterniflora, numerous ducks of the Anitini tribe that sieve seeds (e.g., Black duck (Anus rubripes) and Mallard (Anus platyrhynchos)); marsh generalists: Song sparrow (Melospiza melodia), Red-winged blackbird (Agelaius phoeniceus); and other birds such as: Northern harrier (Circus cyaneus), Short-eared owl (Asio flammeus), Common grackle (Quiscalus quiscula). The relatively high diversity of birds of all other types on the high marsh is considered an "edge effect" of the marsh-upland ecotone where terrestrial birds do mix with birds more typical of the estuary (Nixon 1982).
Mammal Habitat:
A few small mammals are associated directly with the salt marsh, namely, Muskrat (Ondatra zibethicus), meadow vole (Microtus pennsylvanicus), Racoon (Procyon lotor) and shrews (Sorax spp.). Muskrats and voles eat the marsh vegetation and their presence is evidenced by their runways. Muskrats prefer brackish marshes and are common in reed marshes composed of cattails (Typha spp.) and/or common reed (Phragmites australis). Shrews and raccoons prey mainly on small animals, especially invertebrates.
The conservation significance of tidal salt marshes as wildlife habitat are evidenced by the high priority given in the various northeastern state’s “Comprehensive Wildlife Conservation Strategy”. These wildlife action plans proactively assess the health of each state’s wildlife and habitats, identify the problems they face, and outline the actions that are needed to conserve them over the longterm. All 50 States and five U.S. territories developed a State Wildlife Action Plan (SWAP) in 2005 and are expected to update in 2015. To see more and select a state go to http://www.teaming.com/state-wildlife-action-plans-swaps.
Hydrological functions
The hydrology of the salt marsh ecosystem is dominated by tidal exchange with the Atlantic Ocean including Long Island Sound and Narragansett Bay. The trend in the highest astronomical range are in two directions - northward toward ME (Portsmouth, NH, 2.864 m great tidal range [GT]) and east toward New York City (Kings Point, NY 2.378 m great tidal range [GT]), with lessor tidal amplitudes at intervening stations (Wood Hole, MA, ).67 m great tidal range [GT]).
Recreational uses
Many recreational activities, such as boating, hiking, wildlife observation, painting, and tourism including sporting activities like hunting, fishing, and crabbing, provide recreational opportunities for the public as well as economic opportunities for private landowners. Town landings, boat launches, and both public and private parks and beaches are located nearby. Recreational boating, tours and fishing is available throughout the coastal region. Bird watching along the coast has become increasingly popular with the public.
Wood products
n/a
Other products
n/a
Supporting information
Inventory data references
The data contained in this document is derived from the analysis of field inventories (relevé plots and reconnaissance notes collected by MLRA Soil Survey Office 12-TOL. Eighteen high intensity plots were conducted for the reference state during the NRCS halinty Phase II / ESD Project and the NRCS Surface Elevation Table (SET) Monitoring Project. This information was supplemented by plot data from the Connecticut Department of Energy and Environmental Protection (CT-DEEP) Wildlife Unit, CT-DEEP / NRCS Crab Creek Restoration Project, New york Natural Heritage Program retreived through VegBank (http://vegbank.org/vegbank/index.jsp; accessed 7/1/2014) and National Park Service plots collected at Hatches Harbor by the principal author.
Type locality
Location 1: New London County, CT | |
---|---|
Latitude | 41° 17′ 12″ |
Longitude | -72° 19′ 25″ |
General legal description | 2014CT011007 High marsh near state boat ramp, Old Lyme, CT |
Location 2: Middlesex County, CT | |
Latitude | 41° 15′ 29″ |
Longitude | -72° 33′ 26″ |
General legal description | 2014CT009002 High marsh located off service rd at Hammonassett State Park, Madison, CT |
Other references
LITERATURE CITED
Adamowicz, S. C., and C. T. Roman. 2002. Initial Ecosystem Response of Salt Marshes to Ditch Plugging and Pool Creation: Experiments at Rachel Carson National Wildlife Refuge (Maine). Unpubl. Rpt., USGS, Patuxent Wildlife Research Center.
Adamowicz, S., and C. Roman. 2005. New England salt marsh pools: A quantitative analysis of geomorphic and geographic features. Wetlands 25:279–288.
Alber, M., E. M. Swenson, S. C. Adamowicz, and I. A. Mendelssohn. 2008. Salt marsh dieback: an overview of recent events in the US. Estuarine, Coastal and Shelf Science 80:1–11.
Altieri, A. H., M. D. Bertness, T. C. Coverdale, N. C. Herrmann, and C. Angelini. 2012. A trophic cascade triggers collapse of a salt-marsh ecosystem with intensive recreational fishing. Ecology 93:1402–1410.
Amsberry, L., M. A. Baker, P. J. Ewanchuk, and M. D. Bertness. 2000. Clonal integration and the expansion of Phragmites australis. Ecological Applications 10:1110–1118.
Anisfeld, S. C., and T. D. Hill. 2012. Fertilization Effects on Elevation Change and Belowground Carbon Balance in a Long Island Sound Tidal Marsh. Estuaries and Coasts 35:201–211.
Aslam, R., N. Bostan, M. M. Nabgha-e-Amen, and W. Safdar. 2011. A critical review on halophytes: Salt tolerant plants.
Barrett, N. E., and W. A. Niering. 1993. Tidal Marsh Restoration: Trends in Vegetation Change Using a Geographical Information System (GIS). Restoration Ecology 1:18–28.
Bequaert, J. 1943. The genus Littorina in the western Atlantic. Johnsonia 1:1–27.
Bertness, M. D. 1984. Habitat and Community Modification by An Introduced Herbivorous Snail. Ecology 65:370–381.
Bertness, M. D. 1985. Fiddler Crab Regulation of Spartina alterniflora Production on a New England Salt Marsh. Ecology 66:1042–1055.
Bertness, M. D. 1991a. Interspecific Interactions among High Marsh Perennials in a New England Salt Marsh. Ecology 72:125–137.
Bertness, M. D. 1991b. Zonation of Spartina Patens and Spartina Alterniflora in New England Salt Marsh. Ecology 72:138–148.
Bertness, M. D. 1992. The Ecology of a New England Salt Marsh. American Scientist 80:260–268.
Bertness, M. D., and R. Callaway. 1994. Positive interactions in communities. Trends in Ecology & Evolution 9:191–193.
Bertness, M. D., and T. C. Coverdale. 2013. An invasive species facilitates the recovery of salt marsh ecosystems on Cape Cod. Ecology.
Bertness, M. D., C. M. Crain, C. T. Holdredge, and N. C. Sala. 2008. Eutrophication and Consumer Control of New England Salt Marsh Primary Productivity. Conservation Biology 22:131–139.
Bertness, M. D., and A. M. Ellison. 1987. Determinants of Pattern in a New England Salt Marsh Plant Community. Ecological Monographs 57:129–147.
Bertness, M. D., and P. J. Ewanchuk. 2002. Latitudinal and Climate-Driven Variation in the Strength and Nature of Biological Interactions in New England Salt Marshes. Oecologia 132:392–401.
Bertness, M. D., P. J. Ewanchuk, and B. R. Silliman. 2002. Anthropogenic modification of New England salt marsh landscapes. Proceedings of the National Academy of Sciences 99:1395–1398.
Bertness, M. D., L. Gough, and S. W. Shumway. 1992a. Salt tolerances and the distribution of fugitive salt marsh plants. Ecology:1842–1851.
Bertness, M. D., and S. D. Hacker. 1994. Physical Stress and Positive Associations Among Marsh Plants. The American Naturalist 144:363–372.
Bertness, M. D., and G. H. Leonard. 1997. The role of positive interactions in communities: lessons from intertidal habitats. Ecology 78:1976–1989.
Bertness, M. D., and S. W. Shumway. 1993. Competition and Facilitation in Marsh Plants. The American Naturalist 142:718–724.
Bertness, M. D., and B. R. Silliman. 2008. Consumer Control of Salt Marshes Driven by Human Disturbance. Conservation Biology 22:618–623.
Bertness, M. D., and S. M. Yeh. 1994. Cooperative and Competitive Interactions in the Recruitment of Marsh Elders. Ecology 75:2416–2429.
Bertness, M., K. Wikler, and T. Chatkupt. 1992b. Flood tolerance and the distribution of Iva frutescens across New England salt marshes. Oecologia 91:171–178.
Boon, J. D. 2012. Evidence of Sea Level Acceleration at U.S. and Canadian Tide Stations, Atlantic Coast, North America. Journal of Coastal Research:1437–1445.
Bourn, W. S., and C. Cottam. 1950. Some biological effects of ditching tidewater marshes. US Government Printing Office.
Brewer, J. S., J. M. Levine, and M. D. Bertness. 1998. Interactive effects of elevation and burial with wrack on plant community structure in some Rhode Island salt marshes. Journal of Ecology 86:125–136.
Bricker-Urso, S., S. W. Nixon, J. K. Cochran, D. J. Hirschberg, and C. Hunt. 1989. Accretion Rates and Sediment Accumulation in Rhode Island Salt Marshes. Estuaries 12:300–317.
Bromberg, K. D., and M. D. Bertness. 2005. Reconstructing New England salt marsh losses using historical maps. Estuaries 28:823–832.
Bruno, J. F., J. J. Stachowicz, and M. D. Bertness. 2003. Inclusion of facilitation into ecological theory. Trends in Ecology & Evolution 18:119–125.
Buchsbaum, R. N., L. A. Deegan, J. Horowitz, R. H. Garritt, A. E. Giblin, J. P. Ludlam, and D. H. Shull. 2009. Effects of regular salt marsh haying on marsh plants, algae, invertebrates and birds at Plum Island Sound, Massachusetts. Wetlands Ecology and Management 17:469–487.
Buchsbaum, R., I. Valiela, and J. M. Teal. 1981. Grazing by Canada Geese and Related Aspects of the Chemistry of Salt Marsh Grasses. Colonial Waterbirds 4:126–131.
Burdick, D., and C. Roman. 2012. Salt Marsh Responses to Tidal Restriction and Restoration. Pages 373–382 in C. Roman and D. Burdick, editors. Tidal Marsh Restoration. Island Press/Center for Resource Economics.
Cahoon, D. 2006. A review of major storm impacts on coastal wetland elevations. Estuaries and Coasts 29:889–898.
Cahoon, D. R., J. W. Day, Jr., and D. J. Reed. 1999. The influence of surface and shallow subsurface soil processes on wetland elevation: a synthesis. Current Topics in Wetland Biogeochemistry 3:72–88.
Cahoon, D. R., and G. R. Guntenspergen. 2010. Climate change, sea-level rise, and coastal wetlands. Our Changing Climate 32.
Cahoon, D. R., P. F. Hensel, T. Spencer, D. J. Reed, K. L. McKee, and N. Saintilan. 2006. Coastal wetland vulnerability to relative sea-level rise: wetland elevation trends and process controls. Pages 271–292 Wetlands and natural resource management. Springer.
Charles, H., and J. S. Dukes. 2009. Effects of Warming and Altered Precipitation on Plant and Nutrient Dynamics of a New England Salt Marsh. Ecological Applications 19:1758–1773.
Connell, J. H., and R. O. Slatyer. 1977. Mechanisms of Succession in Natural Communities and Their Role in Community Stability and Organization. The American Naturalist 111:1119–1144.
Coverdale, T. C., M. D. Bertness, and A. H. Alteiri. 2013a. Regional Ontogeny of New England Salt Marsh Die-Off. Conservation Biology 27:1041–1048.
Coverdale, T. C., N. C. Herrmann, A. H. Altieri, and M. D. Bertness. 2013b. Latent impacts: the role of historical human activity in coastal habitat loss. Frontiers in Ecology and the Environment 11:69–74.
Cowardin, L. M., V. Carter, F. C. Golet, and E. T. LaRoe. 1979. Classification of Wetlands and Deepwater Habitats of the US. DIANE Publishing.
Crain, C. M., K. Gedan, M. Dionne, B. Silliman, and B. Grosholz. 2009. Tidal restrictions and mosquito ditching in New England marshes. University of California Press: Berkely, CA, USA.
Deegan, L. A., D. S. Johnson, R. S. Warren, B. J. Peterson, J. W. Fleeger, S. Fagherazzi, and W. M. Wollheim. 2012. Coastal eutrophication as a driver of salt marsh loss. Nature 490:388–392.
Donnelly, J. P., and M. D. Bertness. 2001. Rapid shoreward encroachment of salt marsh cordgrass in response to accelerated sea-level rise. Proceedings of the National Academy of Sciences 98:14218–14223.
Donnelly, J. P., S. S. Bryant, J. Butler, J. Dowling, L. Fan, N. Hausmann, P. Newby, B. Shuman, J. Stern, K. Westover, and T. Webb. 2001. 700 yr sedimentary record of intense hurricane landfalls in southern New England. Geological Society of America Bulletin 113:714–727.
Donnelly, J. P., P. Cleary, P. Newby, and R. Ettinger. 2004. Coupling instrumental and geological records of sea-level change: Evidence from southern New England of an increase in the rate of sea-level rise in the late 19th century. Geophysical Research Letters 31:L05203.
Dreyer, G. D., W. A. Niering, and T. R. Ouellette. 1995. Tidal marshes of Long Island Sound: ecology, history and restoration. Connecticut College Arboretum.
Edinger, G. J., D. J. Evans, S. Gebauer, T. G. Howard, D. M. Hunt, and A. M. Olivero. 2002. Ecological Communities of New York State. Second Edition. New York Natural Heritage Program, New York State Department of Envioronmental Conservation, Albany, NY. Ellison, A. 1987. Effects of Competition, Disturbance, and Herbivory on Salicornia Europaea. Ecology 68:576–586.
Elmer, W. H., and R. E. Marra. 2011. New species of Fusarium associated with dieback of Spartina alterniflora in Atlantic salt marshes. Mycologia 103:806–819. Emery, N. C., P. J.
Ewanchuk, and M. D. Bertness. 2001. Competition and salt-marsh plant zonation: stress tolerators may be dominant competitors. Ecology 82:2471–2485.
Enser, R., D. Gregg, C. Sparks, P. August, P. Jordan, J. Coit, C. Raithel, B. Tefft, B. Payton, C. Brown, C. LaBash, S. Comings, and K. Ruddock. 2011. Rhode Island Ecological Communities Classification. Rhode Island Natural History Survey, Kingston, RI.
Erdle, S. Y., J. L. Davis, K. G. Sellner, and Chesapeake Research Consortium. 2008. Management, Policy, Science, and Engineering of Nonstructural Erosion Control in the Chesapeake Bay: Proceedings of the 2006 Living Shoreline Summit. Chesapeake Research Consortium.
Erwin, R. M., J. S. Hatfield, M. A. Howe, and S. S. Klugman. 1994. Waterbird use of saltmarsh ponds created for open marsh water management. The Journal of wildlife management:516–524.
Ewanchuk, P. J., and M. D. Bertness. 2003. Recovery of a Northern New England Salt Marsh Plant Community from Winter Icing. Oecologia 136:616–626.
Ewanchuk, P. J., and M. D. Bertness. 2004. The Role of Waterlogging in Maintaining Forb Pannes in Northern New England Salt Marshes. Ecology 85:1568–1574.
Fagherazzi, S., M. L. Kirwan, S. M. Mudd, G. R. Guntenspergen, S. Temmerman, A. D’Alpaos, J. van de Koppel, J. M. Rybczyk, E. Reyes, C. Craft, and J. Clough. 2012. Numerical models of salt marsh evolution: Ecological, geomorphic, and climatic factors. Reviews of Geophysics 50:RG1002.
Flowers, T. J., M. A. Hajibagheri, and N. J. W. Clipson. 1986. Halophytes. The Quarterly Review of Biology 61:313–337.
Friedrichs, C. T., and J. E. Perry. 2001. Tidal salt marsh morphodynamics: a synthesis. Journal of Coastal Research:7–37.
Gawler, S. C., and A. Cutko. 2010. Natural landscapes of Maine: A guide to natural communities and ecosystems. Maine Natural Areas Program, Department of Conservation.
Gedan, K. B., A. H. Altieri, and M. D. Bertness. 2011. Uncertain future of New England salt marshes. Marine Ecology Progress Series 434:229–237.
Gedan, K. B., and M. D. Bertness. 2009. Experimental warming causes rapid loss of plant diversity in New England salt marshes. Ecology Letters 12:842–848.
Gedan, K. B., B. Silliman, and M. Bertness. 2009. Centuries of human-driven change in salt marsh ecosystems. Annual Review of Marine Science 1:117–141.
Gedan, K., and M. Bertness. 2010. How will warming affect the salt marsh foundation species Spartina patens and its ecological role? Oecologia 164:479–487.
Gosselink, J. G., and R. Baumann. 1980. Wetland inventories: wetland loss along the United States coast. Z. Geomorphol 34:173.
Grime, J. P. 2006. Plant strategies, vegetation processes, and ecosystem properties. John Wiley & Sons. Harper, J. L. 1977. Population biology of plants. Population biology of plants.
Harrison, E. Z., and A. L. Bloom. 1977. Sedimentation rates on tidal salt marshes in Connecticut. Journal of Sedimentary Research 47:1484–1490.
Harshberger, J. W. 1916. The Origin and Vegetation of Salt Marsh Pools. Proceedings of the American Philosophical Society 55:481–484.
Hartig, E., V. Gornitz, A. Kolker, F. Mushacke, and D. Fallon. 2002. Anthropogenic and climate-change impacts on salt marshes of Jamaica Bay, New York City. Wetlands 22:71–89.
Hartman, J. M. 1984. The role of wrack disturbance in the vegetation of a New England salt marsh.
Holdredge, C., M. D. Bertness, and A. H. Altieri. 2009. Role of Crab Herbivory in Die-Off of New England Salt Marshes. Conservation Biology 23:672–679.
Hoover, M., D. Civco, and A. Whelchel. 2010. The development of a salt marsh migration tool and its application in Long Island Sound. San Diego, CA.
IPCC. 2013a. Climate Change 2013: The Physical Science Basis. Contibution of Working Group I to the Fifth Assessment Report of the Intergovermental Panel on Climate Change. http://www.ipcc.ch/report/ar5/.
IPCC. 2013b. Climate Change 2013. The Physical Science Basis. Contribution of Working Group I to the Fifth Assessment Report of the intergovernment Panel on Climate Change. Cambridge University Press, Cambridge, UK and New York, NY, USA.
James-Pirri, M.-J., R. M. Erwin, D. J. Prosser, and J. D. Taylor. 2012. Responses of Salt Marsh Ecosystems to Mosquito Control Management Practices along the Atlantic Coast (U.S.A.). Restoration Ecology 20:395–404.
Jefferies, R. L., A. P. Jano, and K. F. Abraham. 2006. A biotic agent promotes large-scale catastrophic change in the coastal marshes of Hudson Bay. Journal of Ecology 94:234–242.
Kennish, M. J. 2001. Coastal Salt Marsh Systems in the U.S.: A Review of Anthropogenic Impacts. Journal of Coastal Research 17:731–748.
Kirwan, M. L., G. R. Guntenspergen, A. D’Alpaos, J. T. Morris, S. M. Mudd, and S. Temmerman. 2010. Limits on the adaptability of coastal marshes to rising sea level. Geophysical Research Letters 37.
Kirwan, M. L., and J. P. Megonigal. 2013. Tidal wetland stability in the face of human impacts and sea-level rise. Nature 504:53–60.
Koch, F., and C. Gobler. 2009. The Effects of Tidal Export from Salt Marsh Ditches on Estuarine Water Quality and Plankton Communities. Estuaries and Coasts 32:261–275.
Lefor, M., W. Kennard, and D. Civco. 1987. Relationships of salt-marsh plant distributions to tidal levels in Connecticut, USA. Environmental Management 11:61–68.
Levine, J. M., J. S. Brewer, and M. D. Bertness. 1998. Nutrients, Competition and Plant Zonation in a New England Salt Marsh. Journal of Ecology 86:285–292.
Linthurst, R. A. 1979. The Effect of Aeration on the Growth of Spartina alterniflora Loisel. American Journal of Botany 66:685–691.
Mcleod, E., B. Poulter, J. Hinkel, E. Reyes, and R. Salm. 2010. Sea-level rise impact models and environmental conservation: A review of models and their applications. Ocean & Coastal Management 53:507–517.
Metzler,, K. J., and J. P. Barrett. 2006. The Vegetation of Connecticut - A Preliminary Classification. State Geological and Natural History Survey of Connecticut, Department of Environmental Protection, Hartford, CT.
Miller, W. R., and F. E. Egler. 1950. Vegetation of the Wequetequock-Pawcatuck Tidal-Marshes, Connecticut. Ecological Monographs 20:143–172.
Morris, J. T., P. V. Sundareshwar, C. T. Nietch, B. Kjerfve, and D. R. Cahoon. 2002. esponces of coastal wetlands to rising sea level. Ecology 83:2869–2877.
Nichols, G. E. 1920. The Vegetation of Connecticut. VII. The Associations of Depositing Areas Along the Seacoast. Bulletin of the Torrey Botanical Club 47:511–548.
Niering, W. A., and R. S. Warren. 1975. Tidal Wetlands of Connecticut, Vegetation and Micro-Relief. II, pt. 4: Micro-relief. Department of Environmental Protection, State of Connecticut, Hartford. State of CT, Department of Environmental Protection.
Niering, W. A., and R. S. Warren. 1980. Vegetation Patterns and Processes in New England Salt Marshes. BioScience 30:301–307.
Nixon, S. W. 1982. The ecology of New England high salt marshes: a community profile. National Coastal Ecosystems Team, Washington, DC (USA); Rhode Island Univ., Kingston, RI (USA). Graduate School of Oceanography.
NOAA/NOS/CO-OPS. 2014, January 29. Sea Level Trends - NOAA Tides & Currents. http://tidesandcurrents.noaa.gov/sltrends/. Nyman, J. A., and R. H. Chabreck. 1995. Fire in coastal marshes: history and recent concerns.
Odum, W. E. 1988. Comparative Ecology of Tidal Freshwater and Salt Marshes. Annual Review of Ecology and Systematics 19:147–176.
Odum, W. E., T. J. Smith III, J. K. Hoover, and C. C. McIvor. 1984. Ecology of tidal freshwater marshes of the United States east coast: A community profile. Virginia Univ., Charlottesville (USA). Dept. of Environmental Sciences.
Pace, M. L., J. J. Cole, S. R. Carpenter, and J. F. Kitchell. 1999. Trophic cascades revealed in diverse ecosystems. Trends in Ecology & Evolution 14:483–488.
Pennings, S. C., and M. D. Bertness. 1999. Using latitudinal variation to examine effects of climate on coastal salt marsh pattern and process. Current Topics in Wetland Biogeochemistry 3:100–111.
Van de Plassche, O. 1991. Late Holocene Sea-Level Fluctuations on the Shore of Connecticut Inferred from Transgressive and Regressive Overlap Boundaries in Salt-Marsh Deposits. Journal of Coastal Research:159–179.
Portnoy, J. W., and A. E. Giblin. 1997. Biogeochemical effects of seawater restoration to diked salt marshes. Ecological Applications 7:1054–1063.
Potente, J. E. 2007. Geomorphic Alteration of Tidal Wetlands by Mosquito Control Agencies. Unpubl. Rpt., SUNY-Stoney Brook.
Power, M. E. 1992. Top-Down and Bottom-Up Forces in Food Webs: Do Plants Have Primacy. Ecology 73:733–746.
Redfield, A. C. 1972. Development of a New England Salt Marsh. Ecological Monographs 42:201–237.
Reed, D. J. 1995. The response of coastal marshes to sea-level rise: Survival or submergence? Earth Surface Processes and Landforms 20:39–48.
Rochlin, I., M.-J. James-Pirri, S. C. Adamowicz, M. E. Dempsey, T. Iwanejko, and D. V. Ninivaggi. 2012a. The effects of Integrated Marsh Management (IMM) on salt marsh vegetation, nekton, and birds. Estuaries and coasts 35:727–742.
Rochlin, I., M.-J. James-Pirri, S. Adamowicz, R. Wolfe, P. Capotosto, M. Dempsey, T. Iwanejko, and D. Ninivaggi. 2012b. Integrated Marsh Management (IMM): a new perspective on mosquito control and best management practices for salt marsh restoration. Wetlands Ecology and Management 20:219–232.
Roman, C. T. 2012. Tidal marsh restoration: a synthesis of science and management. Island Press.
Roman, C. T., W. A. Niering, and R. S. Warren. 1984. Salt marsh vegetation change in response to tidal restriction. Environmental Management 8:141–149.
Rozsa, R. 1995. Human impacts on tidal wetlands: history and regulations. Tidal Marshes of Long Island Sound: Ecology, History, and Restoration, Connecticut College Arboretum Bull.
Sala, N. M., M. D. Bertness, and B. R. Silliman. 2008. The dynamics of bottom–up and top–down control in a New England salt marsh. Oikos 117:1050–1056.
Sallenger, A. H., K. S. Doran, and P. A. Howd. 2012. Hotspot of accelerated sea-level rise on the Atlantic coast of North America. Nature Clim. Change 2:884–888.
Silliman, B. R., E. D. Grosholz, and M. D. Bertness. 2009. Human Impacts on Salt Marshes: a Global Perspective. niversity of California Press, Berkeley and Los Angeles, CA.
Smith, J. P., and M. Carullo. 2007. Survey of Potential Marsh Dieback Sites in Coastal Massachusetts. Unpubl.
Smith, S. M. 2009. Multi-decadal changes in salt marshes of Cape Cod, MA: photographic analyses of vegetation loss, species shifts, and geomorphic change. Northeastern Naturalist 16:183–208.
Smith, T. J., and W. E. Odum. 1981. The Effects of Grazing by Snow Geese on Coastal Salt Marshes. Ecology 62:98–106.
Sperduto, D. D., and W. F. Nichols. 2012. Natural Communities of New Hampshire Second Edition. NH Natural Heritage Bureau, Concord, NH.
Stumpf, R. P. 1983. The process of sedimentation on the surface of a salt marsh. Estuarine, Coastal and Shelf Science 17:495–508.
Swain, P. C., and J. B. Kearsley. 2012. Classification of the Natural Communities of Massachusetts. Version 1.4. Natural Heritage & Endangered Species Program, Division of Fisheries & Wildlife, Westborough, MA.
Teal, J. M. 1986. The ecology of regularly flooded salt marshes of New England: A community profile. Woods Hole Oceanographic Institution, MA (USA).
Theve, M. C. 2013. Halinity in Tidal Soils of the Connecticut River. University of Connecticut, Storrs, CT.
Thursby, G. B., and M. A. Abdelrhman. 2004. Growth of the Marsh Elder Iva frutescens in Relation to Duration of Tidal Flooding. Estuaries 27:217–224.
Tiner, R. W. 2013. Tidal Wetlands Primer. University of Massachusetts Press.
Tiner, R. W., I. J. Huber, T. Nuerminger, and E. Marshall. 2006. Salt marsh trends in selected estuaries of Southwestern Connecticut. UMASS.
Tonjes, D. J. 2013. Impacts from ditching salt marshes in the mid-Atlantic and northeastern United States. Environmental Reviews 21:116–126.
Turner, R. E. 2011. Beneath the Salt Marsh Canopy: Loss of Soil Strength with Increasing Nutrient Loads. Estuaries and Coasts 34:1084–1093.
Turner, R. E., B. L. Howes, J. M. Teal, C. S. Milan, E. M. Swenson, and D. D. Goehringer-Toner. 2009. Salt marshes and eutrophication: An unsustainable outcome. Limnology and Oceanography 54:1634.
Tyrrell, M. C., M. Dionne, and J. A. Edgerly. 2008. Physical factors mediate effects of grazing by a non-indigenous snail species on saltmarsh cordgrass (Spartina alterniflora) in New England marshes. ICES Journal of Marine Science: Journal du Conseil 65:746–752.
UNESCO. 1981. Background papers and supporting data on the practical salinity scale 1978. Technical Papers in Marine Science 37.
Valiela, I., and C. S. Rietsma. 1995. Disturbance of Salt Marsh Vegetation by Wrack Mats in Great Sippewissett Marsh. Oecologia 102:106–112.
Valiela, I., J. M. Teal, and N. Y. Persson. 1976. Production and dynamics of experimentally enriched salt marsh vegetation: belowground biomass. Limnology and Oceanography 21:245–252.
Varekamp, J. C., and E. Thomas. 1998. Climate change and the rise and fall of sea level over the millennium. Eos, Transactions American Geophysical Union 79:69–75.
Vincent, R., D. Burdick, and M. Dionne. 2013. Ditching and Ditch-Plugging in New England Salt Marshes: Effects on Plant Communities and Self-Maintenance. Estuaries and Coasts:1–15.
Warren, R. S., P. E. Fell, R. Rozsa, A. H. Brawley, A. C. Orsted, E. T. Olson, V. Swamy, and W. A. Niering. 2002. Salt Marsh Restoration in Connecticut: 20 Years of Science and Management. Restoration Ecology 10:497–513.
Warren, R. S., and W. A. Niering. 1993. Vegetation Change on a Northeast Tidal Marsh: Interaction of Sea-Level Rise and Marsh Accretion. Ecology 74:96–103.
Weinstein, M., J. Teal, J. Balletto, and K. Strait. 2001. Restoration principles emerging from one of the world’s largest tidal marsh restoration projects. Wetlands Ecology and Management 9:387–407.
Weston, N. 2014. Declining Sediments and Rising Seas: an Unfortunate Convergence for Tidal Wetlands. Estuaries and Coasts 37:1–23.
Wood, M. E., J. T. Kelley, and D. F. Belknap. 1989. Patterns of Sediment Accumulation in the Tidal Marshes of Maine. Estuaries 12:237–246.
Zhang, L., and H. Shao. 2013. Direct plant–plant facilitation in coastal wetlands: A review. Estuarine, Coastal and Shelf Science 119:1–6.
Contributors
Nels Barrrett Ph.D.
Approval
Greg Schmidt, 10/04/2024
Acknowledgments
Marissa Theve and the tech team acknowledged for compiling the soils and MLRA information.
Nels Barrett, Ph.D. ESI NRCS Tolland
Marissa Theve SS NRCS Tolland
Donald Parizek SSOL NRCS Tolland
Jacob Isleib SS NRCS Tolland
Deb Surabian SSS Tolland
Paul Capatosto CTDEEP Wildlife
Roger Wolfe CTDEEP Wildlife
Rangeland health reference sheet
Interpreting Indicators of Rangeland Health is a qualitative assessment protocol used to determine ecosystem condition based on benchmark characteristics described in the Reference Sheet. A suite of 17 (or more) indicators are typically considered in an assessment. The ecological site(s) representative of an assessment location must be known prior to applying the protocol and must be verified based on soils and climate. Current plant community cannot be used to identify the ecological site.
Author(s)/participant(s) | Nels Barrett, Ph.D. |
---|---|
Contact for lead author | |
Date | 07/03/2014 |
Approved by | Greg Schmidt |
Approval date | |
Composition (Indicators 10 and 12) based on | Foliar Cover |
Indicators
-
Number and extent of rills:
N/A -
Presence of water flow patterns:
Semidiuranal tidal exchange -
Number and height of erosional pedestals or terracettes:
N/A -
Bare ground from Ecological Site Description or other studies (rock, litter, lichen, moss, plant canopy are not bare ground):
Occasional patches (typically less than 30%)of bare ground due to chronic disturbances, e.g., wrack, ice rafting/scouring, infrequent washovers. -
Number of gullies and erosion associated with gullies:
N/A -
Extent of wind scoured, blowouts and/or depositional areas:
Infrequent washovers due to severe coastal storms. -
Amount of litter movement (describe size and distance expected to travel):
20% detrital export (Tiner 2013) - litter size and movement varies from wrack redistribution to detris
-
Soil surface (top few mm) resistance to erosion (stability values are averages - most sites will show a range of values):
Turf-building salt high marsh grasses usually check erosion.
Exposed soil surface suceptable to erosion in proportion to the magnitude of the flood disturbance e.g., greatest in coastal storms. -
Soil surface structure and SOM content (include type of structure and A-horizon color and thickness):
Oe - 0-25 cm very dark gray (10YR 3/1), structureless and massive, 45% SOM -
Effect of community phase composition (relative proportion of different functional groups) and spatial distribution on infiltration and runoff:
N/A salt marsh soils are saturated -
Presence and thickness of compaction layer (usually none; describe soil profile features which may be mistaken for compaction on this site):
N/A -
Functional/Structural Groups (list in order of descending dominance by above-ground annual-production or live foliar cover using symbols: >>, >, = to indicate much greater than, greater than, and equal to):
Dominant:
Warm-season grassesSub-dominant:
Other:
rushes >> forbs > shrubsAdditional:
-
Amount of plant mortality and decadence (include which functional groups are expected to show mortality or decadence):
Perennial grasses will naturally exhibit a minor amount (less than 5%) of scenscence each year. -
Average percent litter cover (%) and depth ( in):
variable - litter accumulation varies with productivity, and degree of tidal export -
Expected annual annual-production (this is TOTAL above-ground annual-production, not just forage annual-production):
1000 to 10,000 lbs/acre (1120 to 11,208 kg/ha) -
Potential invasive (including noxious) species (native and non-native). List species which BOTH characterize degraded states and have the potential to become a dominant or co-dominant species on the ecological site if their future establishment and growth is not actively controlled by management interventions. Species that become dominant for only one to several years (e.g., short-term response to drought or wildfire) are not invasive plants. Note that unlike other indicators, we are describing what is NOT expected in the reference state for the ecological site:
PHAU7 Phragmites australis var. australis common reed -
Perennial plant reproductive capability:
All plants expected to reproduce annually unless disrupted by catastophic events prior to reproductive phase.
Print Options
Sections
Font
Other
The Ecosystem Dynamics Interpretive Tool is an information system framework developed by the USDA-ARS Jornada Experimental Range, USDA Natural Resources Conservation Service, and New Mexico State University.
Click on box and path labels to scroll to the respective text.